ABSTRACT
This chapter reviews how improvements in the sensitivity and specificity of thyroid tests [total and free thyroid hormones (T4 and T3), TSH, thyroid autoantibodies (TRAb, TPOAb, and TgAb) and thyroglobulin (Tg)] have advanced the detection and treatment of thyroid disorders. The strengths and limitations of current methodologies [Radioimmunoassay (RIA), Immunometric assay (IMA) and Liquid Chromatography/Tandem Mass Spectrometry (LC-MS/MS)] are discussed, together with their propensity for analyte-specific and non-specific interferences relating to analyte heterogeneity (TSH, TgAb and Tg), analyte-specific autoantibodies (T4Ab, T3Ab, TSHAb and TgAb) and interferences from heterophile antibodies (HAb) or assay reagents such as Biotin and Rhuthenium. Currently, between-method differences preclude establishing universal thyroid test reference ranges. However, collaborations between the International Federation of Clinical Chemistry (IFCC), the committee for the standardization of thyroid function tests (C-STFT), and the in-vitro diagnostic (IVD) industry are now focused on eliminating these between-method differences.
INTRODUCTION
Figure 1 shows the timeline for improvements in the sensitivity and specificity of thyroid test methodologies made over the last 60 years (1). In the 1950s the only thyroid test available was an indirect estimate of the serum total (free + protein-bound) thyroxine (T4) concentration, using the protein bound iodine (PBI) technique (2). Early technological advances in radioimmunoassay (RIA) (3-6), immunometric assay (IMA) (7-11), and most recently, liquid chromatography-tandem mass spectrometry (LC-MS/MS) methodologies (12-14) have progressively improved the sensitivity and specificity of thyroid tests. Currently, most thyroid testing is made on serum specimens using automated IMA methodology to measure total thyroid hormones (TT4 and TT3), estimate free thyroid hormones (FT4 and FT3) (13,15,16), and measure TSH (13) and thyroglobulin (Tg) (14,17). Automated IMA methodology is also used to detect autoantibodies that target the TSH receptor (TRAb) (18-20), the thyroid peroxidase enzyme (TPOAb) (21), and the thyroglobulin protein (TgAb) (22-24). When indicated, the thyroid hormone binding proteins thyroxine binding globulin (TBG), transthyretin (TTR)/prealbumin (TBPA), and albumin can also be measured (25-27). The IFCC and CDC continue their efforts to encourage test manufacturers to identify the causes of, and reduce the magnitude of, between-method variability in thyroid hormone and TSH measurements (12,28-33). Isotope-dilution liquid chromatography/tandem mass spectrometry (ID-LC-MS/MS) has become the reference measurement procedure (RMP) for total thyroid hormone measurements (28) and free hormone (FT4 and FT3) measurement in equilibrium dialysates (15,28,34,35). TSH methods are now being re-standardized to the new International Reference Preparation (81/615) and harmonized to the all-method mean (13,33,36). Although serum Tg can now be detected by LC-MS/MS as tryptic peptides (37-42), the clinical value relative to the expense of this technique is still debated (14,41,43). Thus, despite technical improvements in sensitivity, specificity and standardization, the problem of substantial between-method variabilities remains for all tests (13,14,28,30,32,33,35,44-46). Establishing universal thyroid test reference ranges that would apply to all methods, by removing current between-method biases, would greatly benefit healthcare systems worldwide. Current guidelines for managing pregnant (47,48) and non-pregnant patients with hypothyroidism (49-52), hyperthyroidism (53,54), thyroid nodules (55), or differentiated thyroid cancers (DTC) (17,23,56-60) are also referenced.
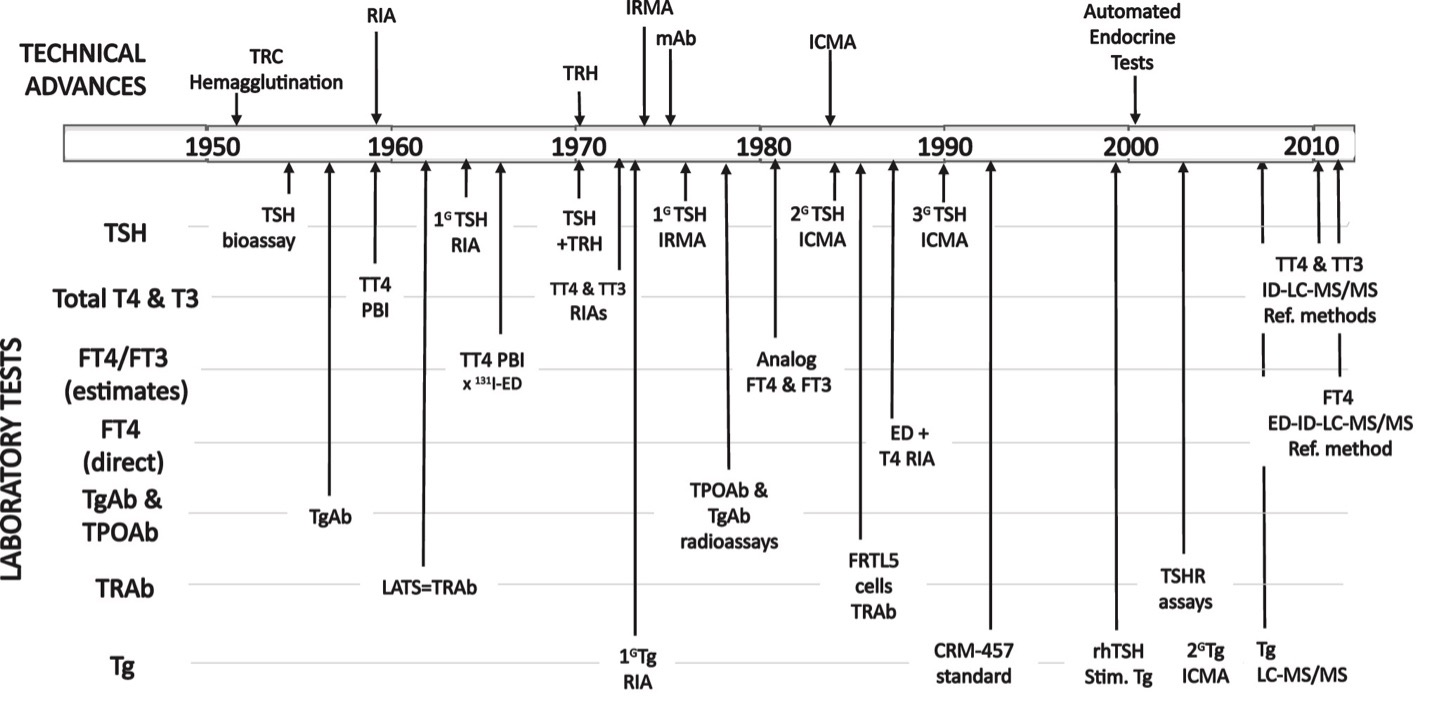
Figure 1. Timeline for the Major Technical Advances in Thyroid Testing. The figure shows the development of increasingly more sensitive TSH tests: first generation, (1G), second generation (2G), and third generation (3G), and advances in the methodologies used to measure total thyroid hormones (TT4 and TT3), indirectly estimate free thyroid hormones (FT4 and FT3), directly measure FT4, and measure the thyroid autoantibodies TPOAb, TgAb, and TRAb and Thyroglobulin (Tg). From reference 1.
TOTAL THYROID HORMONE MEASUREMENTS (TT4 and TT3)
Thyroxine (T4) circulates 99.97 percent bound to the plasma proteins, primarily TBG (60-75 %) but also transthyretin TTR/TBPA (15-30 %) and albumin (~10 %) (25,26,61). In contrast 99.7 % of Triiodothyronine (T3) is bound to TBG (26,61). The total (free + protein-bound) thyroid hormones (TT4 and TT3) circulate at nanomolar concentrations that are considerably easier to measure than the free hormone moieties (FT4 and FT3) that circulate in the picomolar range (62). Serum TT4 methods have evolved over the past five decades from protein-bound iodine and competitive protein binding tests (2,63) to non-isotopic immunometric assays and most recently, isotope dilution tandem mass spectrometry (ID-LC-MS/MS) methods (13,64,65) (66). Since total thyroid hormone concentrations are influenced by conditions that change the binding protein concentrations (Figure 2), the measurement of the free thyroid hormone is considered more clinically reliable (13).
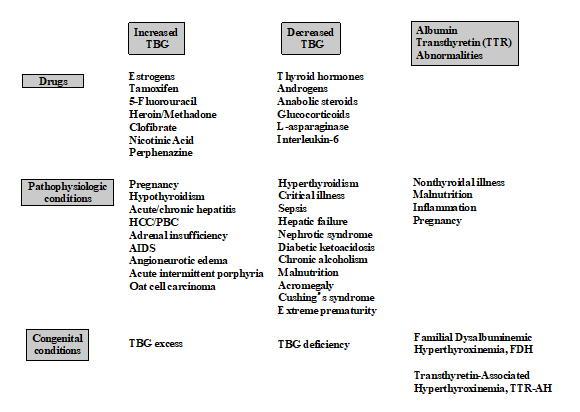
Figure 2. Conditions that Influence Thyroid Hormone Binding Proteins. From references 25, 27 and 61.
Total thyroid hormone methods typically require the inclusion of inhibitors, such as 8-anilino-1-napthalene-sulphonic acid to block hormone binding to serum proteins and facilitate the binding of thyroid hormone to the antibody reagent(s) (67). T3 concentrations are ten-fold lower than T4, so measuring T3 has always presented a greater sensitivity and precision challenge than measuring T4. Currently both TT4 and TT3 are measured by immunometric assays performed on automated platforms using enzymes, fluorescence, or chemiluminescent molecules as signals (11,13,62).
Between-method variability among eleven TT4 and twelve TT3 immunoassays are shown in Figure 3 (28) from sera from healthy individuals and compared with values reported by isotope dilution tandem mass spectrometry (ID-LC-MS/MS) - the reference measurement procedure (RMP) that uses primary T4 and T3 standards for calibration (13,28). Although most methods fell short of the optimal 5 percent goal established by the C-STFT, 4/11 TT4 assays agreed within 10 percent of the reference, whereas most TT3 assays exhibited a positive bias that would necessitate re-standardization (28,68,69). Thus, as would be expected, TT4 assays are more reliable than TT3 assays. However, variability persists likely resulting from matrix differences between calibrators and patient sera, the efficiency of the blocking agent employed, and reagent lot-to-lot variability (13,69-72).
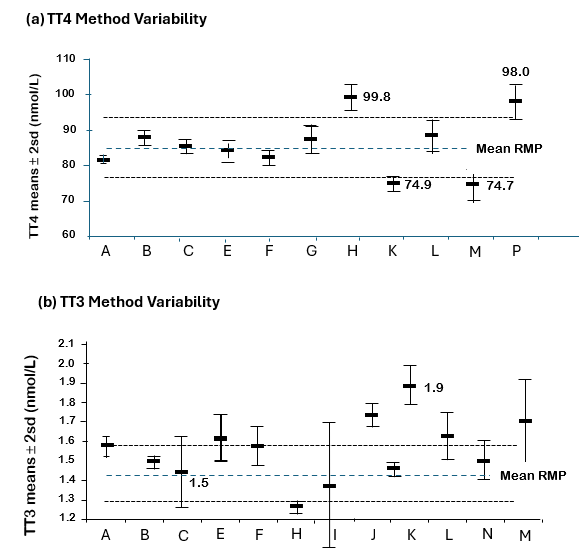
Figure 3. (a) TT4 and (b) TT3 Between-Method Variability. The figure shows the variability among 11 TT4 (A-P) and 12 TT3 (A-M) methods (shown as assay means 2 sd) relative to the RMP for the method. For the assays differing >10% from the RMP mean, the numerical value of the mean is listed (28).
TT4 and TT3 Reference Ranges
The problem of between-method differences in TT4 and TT3 measurements (Figure 3) is compounded by the continued use of non-SI units by some countries. TT4 reference ranges have approximated 58 to 160 nmol/L (4.5-12.5 µg/dL) for more than four decades. However, in euthyroid pregnant women there is an approximate 2-fold rise in TBG concentrations by mid-gestation that produce a steady TT4 increase beginning in the first trimester and plateauing at approximately 1.5-fold pre-pregnancy levels by mid-gestation (73-75). As a result, some have suggested that the non-pregnant TT4 reference range be adjusted by a factor of 1.5 when assessing thyroid status in the latter half of gestation (47,73,74,76). TT3 reference ranges generally approximate 1.2 - 2.7 nmol/L (80 –180 ng/dL) (77), but as shown in Figure 3, TT3 displays more between-method variability than TT4 (69,78).
FREE THYROID HORMONE TESTS (FT4 and FT3)
In accordance with the free hormone hypothesis, it is the free thyroid hormone fractions (0.02 % of TT4 and 0.2 % of TT3) that exert biologic activity at the cellular level (79) and protein-bound hormone is considered biologically inert. Since binding-protein abnormalities are highly prevalent (Figure 2) (25,27,61), free hormone measurements (FT4 and FT3) are preferable to total hormone (TT4 and TT3) (13,15). However, the measurement of free hormone concentrations independent of protein-bound hormone remains technically challenging (13,15,80). This is especially the case for FT3, because FT3 immunoassays are more susceptible to interference by free fatty acids and drugs present in the circulation, prompting many laboratories to prefer a TT3 over a FT3 assay (13). FT4 and FT3 fall into two categories – direct methods that employ a physical separation of free from protein-bound hormone and indirect free hormone estimate tests (16).
Direct FT4 and FT3 Methods
Direct free hormone methods have employed equilibrium dialysis (ED) (13,81,82), ultrafiltration (83-85), or gel filtration (86) to separate free hormone from the dominant protein-bound moiety. The IFCC has now established equilibrium dialysis, isotope dilution, liquid chromatography, tandem mass spectrometry (ED ID-LC-MS/MS) using primary calibrators as the RMP for FT4 measurements (13,32,87-89). Specifically, equilibrium dialysis of serum is performed under defined conditions before measuring FT4 in the dialysate by ID-LC-MS/MS (12,34,35). Manufacturers are recommended to use this RMP to recalibrate their FT4 immunoassay tests (13). However, even direct methods that employ equilibrium dialysis or ultrafiltration to separate free from protein-bound hormone are not immune from technical problems relating to dilution, adsorption, membrane defects, temperature, the influence of endogenous binding protein inhibitors, fatty acid formation, and sample-related effects (13,80,82,90). Because direct free hormone methods are technically demanding, inconvenient, and expensive, they are typically only readily available in reference laboratories and most clinical laboratories use FT4 and FT3 estimate tests - immunoassay “sequestration” methods (see below). However, a direct free hormone test can be especially useful for evaluating thyroid status when immunoassay values appear discordant with the clinical presentation and/or the TSH measurement (15,91). All current FT4 and FT3 estimate tests remain binding-protein dependent to some extent (69).
EQUILIBRIUM DIALYSIS (ED)
Early equilibrium dialysis methods used I131 and later I125 labeled T4 tracers to measure the free T4 fraction, that when multiplied by a total hormone measurement gave an estimate of the free hormone concentration (81). Subsequently, symmetric dialysis in which serum was dialyzed without dilution (or employing a near-physiological medium) was used to overcome dilution effects (82). By the early 1970s higher affinity T4 antibodies (>1x1011 L/mol) and high specific activity T4-I125 tracers were used to develop sensitive RIA methods that could directly measure FT4 and FT3 in dialysates and ultrafiltrates (83,92). Subsequent improvements have involved employing more physiological buffer diluents and improving the dialysis cell design (82,92). More recently, isotope-dilution liquid chromatography/tandem mass spectrometry (ID-LC-MS/MS) (93) has been used to measure FT4 in ultrafiltrates (94) and dialysates (13,32,35,36,87,95,96).
ULTRAFILTRATION METHODS
Ultrafiltration has also been used to remove protein-bound T4 prior to LC-MS/MS measurement of FT4 in the ultrafiltrate (97). Direct FT4 measurements employing ultrafiltration are sometimes higher than those made by equilibrium dialysis, because ultrafiltration avoids dilution effects (98). Moreover, ultrafiltration is not influenced by dialyzable inhibitors of T4-protein binding that can be present in conditions such as non-thyroidal illness (NTI) (90). However, ultrafiltration can be prone to errors when there is a failure to completely exclude protein-bound hormone and/or adsorption of hormone onto the filters, glassware, and tubing (99). In addition, ultrafiltration is temperature dependent such that ultrafiltration performed at ambient temperature (25°C) will report FT4 results that are 67 percent lower than ultrafiltration performed at 37°C (97). However, FT4 concentrations measured by ID-LC-MS/MS following either ultrafiltration at 37°C or equilibrium dialysis usually correlate (100).
GEL ABSORPTION METHODS
Some early direct FT4 methods used Sephadex LH-20 columns to separate free from bound hormone before eluting the free T4 from the column for measurement by a sensitive RIA. However, because of a variety of technical issues, assays based on this methodologic approach are not currently used (62).
Indirect Free T4 and Free T3 Estimate Tests
The first free hormone estimate tests were free hormone “indexes” (FT4I and FT3I) – a correction of the total hormone concentration for the influence of binding proteins assessed either using a direct TBG measurement or a binding-protein estimate (uptake) test (101,102). Current free hormone estimate tests are typically automated immunoassays that employ an antibody to sequester a small amount of the total hormone that is purportedly proportional to the free hormone concentration (13,15). Both index tests (FT4I and FT3I) and FT4 and FT3 immunoassays are typically protein-dependent to some extent and may under- or overestimate free hormone when binding proteins are grossly abnormal (80,103-105). As with TT4 methods, current FT4 immunoassays have significant between-method variability and biases (relative to the RMP) that far exceed the biological FT4 variation (Figure 4) (13,28,69). Recalibrating methods against the RMP has been shown to significantly reduce biases (32). It is hoped that manufacturers will continue to work to eliminate between-method biases and establish reference intervals that would apply to all methods (106).
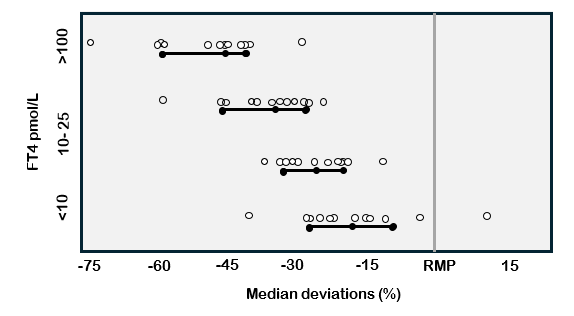
Figure 4. FT4 Between-Method Variability in FT4 Immunoassays. This figure shows deviations in FT4 measurements made by 13 different immunoassays relative to the reference measurement procedure (RMP = ED-ID-LC-MS/MS) (89).
TWO TEST INDEX METHODS (FT4I AND FT3I)
Free hormone indices (FT4I and FT3I) are unitless mathematical calculations made by correcting the total hormone test result for the influence of binding proteins, primarily TBG (107). These indexes that have been used for more than 50 years require two separate tests to estimate free hormone (80). The first test involves the measurement of total hormone (TT4 or TT3) whereas the second test assesses the binding protein concentration by either a direct TBG immunoassay (103), a Thyroid Hormone Binding Ratio (THBR) or “Uptake” test (102), or an isotopic determination of the free hormone fraction (80,108).
TBG Immunoassays
Data has been conflicting concerning whether indexes that employ THBR in preference to a direct TBG are diagnostically superior (109). Free hormone indexes calculated using TBG measurement (TT4/TBG) may offer improved diagnostic accuracy over THBR when the total hormone concentration is abnormally high (i.e. hyperthyroidism), or when drug therapies interfere with THBR tests (110). Regardless, the TT4/TBG index is not totally independent of the TBG concentration, nor does it correct for albumin or transthyretin binding protein abnormalities (figure 2) (104).
Thyroid Hormone Binding Ratio (THBR) / "Uptake" Tests
The first "T3 uptake" tests developed in the 1950s employed the partitioning of T3-I131 tracer between the plasma proteins in the specimen and an inert scavenger (red cell membranes, talc, charcoal, ion-exchange resin, or antibody) (111-113). The "uptake" of T3 tracer onto the scavenger provided an indirect, reciprocal estimate of the TBG concentration in the specimen. Initially, T3 uptake tests were reported as percent uptakes (free/total tracer). Sera with normal TBG concentrations typically had approximately 30 percent of the T3 tracer taken up by the scavenger. During the 1970s methods were refined by replacing I131-T3 tracers by I125-T3 with a calculation of the hormone uptake based on the ratio of isotopic counts between the absorbent, and total minus absorbent counts. Results were expressed as a ratio with normal sera having an assigned value of 1.00 (108). Historically, the use of T3 as opposed to T4 tracer was made for practical reasons relating to the ten-fold lower affinity of TBG for T3 versus T4, facilitating a higher percentage of T3 tracer binding to the scavenger, thereby allowing shorter isotopic counting times. Because current methods use non-isotopic proprietary T4 or T3 "analogs", counting time is no longer an issue and current tests may use a "T4 uptake" approach - which may be more appropriate for correcting for T4-binding protein effects. Differences between T3 and T4 "uptakes" have not been extensively studied (114). Although all THBR tests are to some degree TBG dependent, the calculated FT4I and FT3I usually provides an adequate correction for mild TBG abnormalities (i.e. pregnancy and estrogen therapy) (73,102,103,115) but may fail to correct for grossly abnormal binding proteins (26) seen in euthyroid patients with congenital TBG extremes (103,104,116), familial dysalbuminemias (62,105,117-119), thyroid hormone autoantibodies (120-122), or medications that directly or indirectly influence thyroid hormone binding to plasma proteins (13,62,104,123).
Isotopic Index Methods
The first free hormone tests developed in the 1960s were indexes calculated from the product of the free hormone fraction, measured isotopically by dialysis, and TT4 measured by PBI and later RIA (81). These early isotopic detection systems were technically demanding and included paper chromatography, electrophoresis, magnesium chloride precipitation, and column chromatography (81,124-126). The free fraction index approach was later extended to ultrafiltration (83,85) and symmetric dialysis (127), the latter measuring the rate of transfer of isotopically labeled hormone across a membrane separating two chambers containing the same undiluted specimen. Ultrafiltration and symmetric dialysis had the advantage of eliminating dilution effects that influenced tracer dialysis values (82,128). However, free hormone indexes calculated using an isotopic free fraction were not completely independent of the TBG concentration and were influenced by tracer purity and the buffer matrix employed (92,129).
Clinical Utility of Two-Test Index Methods (FT4I and FT3I)
In the past some have favored the two-test FT4I approach for evaluating the thyroid status of patients with abnormal binding protein states like pregnancy or NTI (73,82). However, the continued use of these FT4I tests remains controversial (130). Until FT4 immunoassays are re-standardized to remove biases (13,69), FT4I remains a useful confirmatory test when binding proteins are abnormal or for diagnosing central hypothyroidism (69).
Free Thyroid Hormone Immunoassay Methods (FT4 and FT3)
Currently, most free hormone testing is made using automated FT4 and FT3 immunoassays (62,131). These immunoassays are based on "one-step", "labeled antibody" or "two-step" principles (80). For more than twenty years controversy has surrounded the standardization and diagnostic accuracy of these methods, especially in pathophysiologic conditions associated with the binding protein abnormalities such as pregnancy (15,73,131). These assays are subject to variability due to polymorphisms, drug interactions, high free fatty acid (FFA) levels, or thyroid binding inhibitors such as those present in non-thyroidal illness (NTI) (11,30, 62, 69, 90, 99,104,105,121,132). Studies of the inverse FT4/TSH log/linear relationship have emphasized the need to evaluate each method with clinical specimens containing abnormal binding proteins (94,133,134). Currently, most FT4 and FT3 immunoassays display significant negative or positive biases that exceed the intra-individual biological variability (12,13). As shown in Figure 4, all but one of the FT4 immunoassays tested had a negative bias relative to the FT4 RMP. Although the IVD industry is being encouraged to recalibrate their free hormone immunoassays against the RMP to reduce between-method biases (13, 28, 69, 87,135), implementation of a global re-calibration effort has been delayed by cost as well as practical, educational, and regulatory complexity.
ONE-STEP FT4 AND FT3 METHODS
The “one-step” approach uses a proprietary labeled hormone analog, designed for minimal interaction with thyroid hormone binding proteins, that competes with hormone in the specimen for a solid-phase anti-hormone antibody in a classic competitive immunoassay format (15,62,80). After washing away unbound constituents, the free hormone concentration should be inversely proportional to the labeled analog bound to the solid support. Although conceptually attractive, the diagnostic utility of the one-step approach has been shown to be dependent on the degree that the analog is "inert" with respect to binding proteins (80,94,133,134).
LABELED ANTIBODY FT4 AND FT3 METHODS
Labeled antibody methods are "one-step" methods that use a labeled antibody in preference to a labeled hormone analog. The free hormone in the specimen competes with solid-phase hormone for the labeled antibody and is quantified as a function of the fractional occupancy of hormone-antibody binding sites in the reaction mixture (15,62,80,136). The labeled antibody approach is used as the basis for several automated immunoassay platforms because it is easy to automate and considered less binding-protein dependent than the labeled analog approach, since the solid phase hormone does not compete with endogenous free hormone for hormone binding proteins (15,80,137-139).
TWO-STEP, BACK TITRATION FT4 AND FT3 METHODS
The two-step approach was first developed by Ekins and colleagues in the late 1970s (79,113). Two-step methods typically employ immobilized T4 or T3 antibody (for FT4 and FT3 immunoassays, respectively) to sequester a small proportion of total hormone from a diluted serum specimen without disturbing the original free to protein-bound equilibrium (62,80). After removing unbound serum constituents by washing, a labeled probe (originally 125-I T4, or more recently a macromolecular T4 conjugate) is added to quantify unoccupied antibody-binding sites that are inversely related to the free hormone concentration - a procedure that has been referred to as "back-titration (80).
CLINICAL UTILITY OF FT4 AND T3 IMMUNOASSAY MEASURMENTS
Current reference ranges for FT4 and FT3 immunoassays are method-dependent because of calibration biases that preclude establishing a universal reference range that would apply across methods (13,68,86). These biases are evident for FT4 immunoassay methods shown in Figure 4. Most FT4 methods give diagnostically reliable results when binding proteins are near-normal, provided that a method-specific reference range is employed (69). However, both TT3 and FT3 immunoassays tend to be inaccurate in the low range (78,140) and have no value for diagnosing or monitoring treatment for hypothyroidism (52,141), although FT3 measurements can be useful for diagnosing or confirming unusual cases of hyperthyroidism.
Ambulatory Patients
FT4 and FT3 tests are used in preference to TT4 or TT3 measurements because they have better diagnostic accuracy for detecting hypo- and hyperthyroidism in patients with abnormal thyroid hormone binding proteins (figure 2). FT4 typically serves as a second-line test for confirming primary thyroid dysfunction detected by an abnormal TSH, but is the first-line test when thyroid status is unstable (early phase of treating hypo- or hyperthyroidism); in the presence of pituitary/hypothalamic disease (when TSH is unreliable); or when patients are taking drugs such as dopamine or glucocorticoids that are known to affect TSH secretion (10,104,110,142). Mild "subclinical" thyroid dysfunction is characterized by a TSH/FT4 discordance (abnormal TSH/normal FT4) reflecting the intrinsic complex nature of the inverse log/linear TSH/FT4 relationship (8,10,143) - a relationship that is modified by age and sex (144,145). Thus, small changes in FT4, even within normal limits, are expected to produce a mild degree of TSH abnormality - between 0.05 and 0.3 mIU/L (with subclinical hyperthyroidism) and 5 and 10 mIU/L (with subclinical hypothyroidism). An unexpected TSH/FT4 discordance if confirmed, should prompt an investigation for interference with FT4, TSH or both tests (91,146,147). FT4 interference can result from severe binding protein abnormalities such as congenital TBG excess or deficiency (26,62,103,148,149), dysalbuminemias (105,150-152), thyroid hormone autoantibodies (147,153-155), or drug interferences (62,104,123).
Pregnant Patients
Current reference ranges for FT4 immunoassays are method-dependent because of calibration biases that precludes establishing a universal reference range that would apply across methods (Figure 4) (156,157). This between-method variability has profound effects on the setting of the FT4 reference range for pregnancy (Figure 5). As with non-pregnant patients, TSH is the first-line test to use for assessing thyroid status during pregnancy (48,158). However, FT4 measurement is needed for monitoring anti-thyroid drug treatment of hyperthyroid pregnant patients who have an undetectable TSH. The question whether an isolated low FT4 during pregnancy is a maternal or fetal risk factor, remains controversial (159,160), although some studies suggest that low FT4 may be a risk factor for gestational diabetes and fetal complications (161-163). Non-pregnant FT4 reference ranges do not apply to pregnancy since FT4 progressively declines as gestation progresses, necessitating the use of a trimester-specific reference ranges (73,158,164,165). Setting universal trimester-specific FT4 reference ranges is currently hampered by the between-method differences shown in Figure 4 and 5 (69,156,165), compounded by the differences related to ethnicity (166-170), iodine intake (171-173), smoking (174), and BMI (145,166). Establishing institution-specific trimester-specific reference ranges from the 2.5 to 97.5 percentiles by recruiting at least 400 pregnant patients (170) is not practical for most institutions. After the proposed re-standardization of FT4 methods against the RMP the feasibility of establishing universal trimester-specific reference ranges will improve (13,69,135). However, binding protein effects will remain, and population-specific factors will still have to be considered.
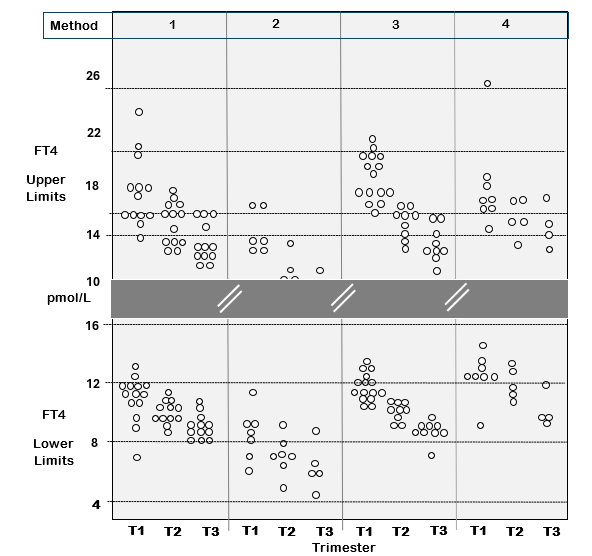
Figure 5. Between-Method FT4 Variability Impacts Thyroid Testing in Pregnancy. The figure shows the upper and lower FT4 reference limits (2.5–97.5%) from 43 published studies of FT4 measurements made in each trimester of pregnancy by four different methods: Abbott (1), Beckman (2), Roche (3) and Siemens (4). The data shows the expected trend for higher FT4 in the first trimester, resulting from thyroidal human chorionic gonadotropin (HCG) stimulation which is maximal in early pregnancy. The data is re-drawn with permission from reference 156.
Hospitalized Patients with Nonthyroidal Illnesses (NTI)
The diagnostic performance of current FT4 methods has not been evaluated in hospitalized patients with NTI where the severity of illness, binding protein inhibitors, and drug therapies can negatively impact the reliability of both thyroid hormone and TSH testing (10,30,62,90,122,132,181-183). Three categories of hospitalized patients deserve special attention: a) patients with NTI without known thyroid dysfunction who have a high or low T4 status; b) patients with primary hypothyroidism and concurrent NTI and, c) patients with hyperthyroidism and concurrent NTI (13). Because the diagnostic reliability of FT4 testing is still questionable in sick hospitalized patients, a combination of both T4 (FT4 or TT4) and TSH may be needed to assess thyroid status in this setting (10,13).
In most clinical situations where FT4 and TSH results are discordant, the TSH test is the most diagnostically reliable, provided that the patient does not have pituitary failure or receiving medications such as glucocorticoids or dopamine that directly inhibit TSH secretion (110,142,181). Repetitive TSH testing may be helpful in resolving the cause of an abnormal FT4, because the TSH abnormalities of NTI are typically transient (Figure 6b) whereas the TSH abnormality will persist if due to underlying thyroid dysfunction (184-187). In some cases, it may be useful to test for TPOAb as a marker for underlying thyroid autoimmunity.
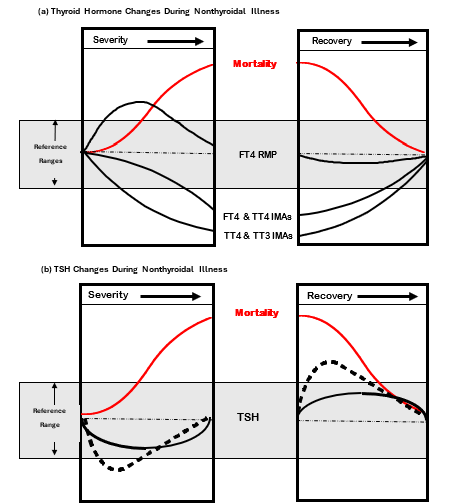
Figure 6. Effects of Nonthyroidal Illness (NTI) on Thyroid Tests. Figure 6a shows the magnitude and direction of changes in total (TT4 and TT3) and free (FT4 and FT3) thyroid hormone IMA tests versus FT4 measured by the RMP (ED-ID-LC-MS/MS), as the severity of illness increases, followed by recovery. Figure 6b shows the magnitude and direction of TSH changes as the severity of illness increases, followed by recovery. Data redrawn from reference 188 with permission.
Pediatric Patients
The determination of normal reference limits for pediatric age groups is especially challenging, given the limited number of studies involving large numbers of healthy children (175-177). Most studies report that serum TSH peaks after birth and steadily declines throughout childhood to reach adult levels at puberty. Likewise, FT3 declines across the pediatric age groups during childhood and approaches the adult range at puberty, whereas FT4 levels for infants less than a year old are higher than for children 1 to 18 years old who have FT4 comparable to adults (175-180).
Interferences with Thyroid Hormone Tests
Only the ordering physician can suspect interference with a test result and request that the laboratory perform interference checks. This is because the hallmark of interference is discordance between the test result and the clinical presentation of the patient, and most specimens are sent to the laboratory with no clinical information. Failure to recognize interferences can have adverse clinical consequences (91,146,189-197).
Laboratory checks for interferences include, a) showing a discordance between different manufacturers methods (196,198-200), b) re-measurement of the analyte after adding a blocker of Heterophile antibodies (HAb) (196,200,201), c) performing linearity studies or d) precipitating interfering immunoglobulins with polyethylene glycol (PEG) (196,198). A change in the analyte concentration in response to any one of these maneuvers suggests interference, but a lack of an effect does not rule out interference.
Interferences can be classified as either (a) non-analyte-specific, or (b) analyte-specific (191,195,199).
NON-ANALYTE SPECIFIC INTERFERENCES
Protein Interferences
Either paraproteins or abnormal immunoglobulins can interfere with immunoassays (90,202-205).
Congenital TBG excess or deficiency: Free hormone immunoassays and free T4 index tests may be susceptible to interference from grossly abnormal TBG concentrations, such as seen in congenital TBG excess or deficiency states (26,62,103,148,149).
Pregnancy: Estrogen stimulation increases TBG, and consequently both TT4 and TT3, concentrations progressively rise to plateau at 2.5-fold pre-pregnancy values by mid-gestation (73). Despite the rise in total hormone, both FT4 and FT3 decline during gestation, in accordance with the law of mass action (73,157,158,206,207). However, the degree of FT4 decline during pregnancy is variable and method-dependent (Figure 5). The declining albumin concentrations typical of late gestation also affect some methods (208).
Familial Dysalbuminemias and Transthyretin Hyperthyroxinemias: Autosomal dominant mutations in the albumin or transthyretin (prealbumin) gene (209) can result in altered protein structures with enhanced affinity for thyroxine and/or triiodothyronine. These abnormal proteins can interfere with FT4 and/or FT3 measurements and result in inappropriately high FT4 and/or FT3 immunoassay values (105,151,210-212). Familial Dysalbuminemic Hyperthyroxinemia (FDH) is a rare condition with a prevalence of ~1.8 percent in the Hispanic population (119,213). It arises from a few genetic variants in the albumin gene, with the R218H being the most common. Some variants result in extremely high TT4, whereas other mutations (i.e. L66P) affect mainly TT3 (150). Affected individuals are euthyroid and have normal TSH and FT4 when measured by direct techniques such as equilibrium dialysis (105). Unfortunately, most FT4 estimate tests (immunoassays and indexes) report falsely high values for FDH patients that may prompt inappropriate treatment for presumed hyperthyroidism if the condition is not recognized (105,119).
Heterophile Antibodies (HAb)
It is well recognized that heterophile antibodies (HAb) - human poly-specific antibodies targeting animal antigens, can interfere with immunometric assays causing falsely high/positive or falsely low/negative test results (214,215). The most common interferant is human anti-mouse antibodies (HAMA) (199,215-220). Rheumatoid factor (RF), an immunoglobulin commonly associated with autoimmune conditions, is also considered a heterophile antibody that can interfere by targeting human antigens (199,217,221,222). Although HAb usually causes false positive tests, false-negative tests have also been reported (214). HAb has been shown to interfere with multiple endocrine tests that use IMA principles, including free and total thyroid hormones, TSH, Tg, and TgAb (138,193,200,214,221,223-225). The prevalence of HAbs is variable but has been reported as high as eleven percent (223,226,227). In recent years assay manufacturers have increased the immunoglobulin blocker reagents added to their tests and this has reduced HAb interference somewhat (223,225-227). However, interference is still seen in some patients with a high enough HAb to overcome the assay blocker (198,223,228). HAb interference mostly affects non-competitive immunometric assays (IMA) that employ monoclonal antibodies of murine origin (216). Assays based on the competitive format that employ high affinity polyclonal antibody reagents, are rarely affected (216). The test marketed by one manufacturer can be severely affected, whereas the test from a different manufacturer may appear unaffected (200). This is why the first step for investigating interference is re-measurement of the analyte by a different method. It should be noted that patients receiving recent vaccines, blood transfusions, or monoclonal antibodies (given for treatment or scintigraphy), as well as veterinarians and those in contact with animals, are especially prone to test interferences caused by induced HAb and human anti-mouse antibodies (HAMA) (198,229).
Anti-Reagent Antibodies
Interference can be caused by antibodies targeting assay reagents. For example, a number of reports have found that anti-rhuthenium antibodies can interfere with TSH, FT4, and FT3 tests (200,230). In addition, antibodies targeting either streptavidin (231,232) or Biotin (233,234) can interfere with assays employing streptavidin or biotin reagents.
High Dose Dietary Biotin
Some IMA tests have employed a biotin-streptavidin separation system (232). Patients who take a high dose of dietary biotin risk having test interferences with such methods (232). Depending on the specific test formulation, biotin interference can cause falsely high- or low- test results (234-236). Manufacturers are now prioritizing replacing their biotin-streptavidin separation systems to eliminate this problem (237).
ANALYTE-SPECIFIC INTERENCES
Analyte-specific interferences typically result from autoantibodies targeting the analyte (238). Autoantibodies targeting both TSH (macro-TSH) (238-241) and both thyroid hormones (T4 and/or T3) (154,155) have been reported. Autoantibody interferences may be more prevalent in patients with non-thyroid autoimmune conditions (242,243). Depending on the analyte and test formulation, thyroid hormone and TSH autoantibodies typically cause falsely high tests (239,244). It should be noted that transplacental passage of either HAb or anti-analyte autoantibodies (i.e. TSHAb or T4Ab) have the potential to interfere with neonatal screening tests (245-247). Specifically, maternal TSH autoantibodies can cross the placenta and cause a falsely high TSH screening test in the newborn mimicking congenital hypothyroidism (247), whereas maternal T4 autoantibodies could cause a falsely high neonatal T4 test and mask the presence of congenital hypothyroidism (246).
Thyroid Hormone Autoantibodies (T4Ab/T3Ab)
T4 and T3 autoantibodies can falsely elevate total hormone, free hormone, or THBR measurements depending on the method employed (153,155,210). The prevalence of thyroid hormone autoantibodies occurs in approximately 2 percent of the general population but may be present in over 30 percent of patients with autoimmune thyroid disease or other autoimmune conditions (242,243,248). However, despite their high prevalence, significant interference caused by thyroid autoantibodies is not common and depends on the qualitative characteristics of the autoantibody present (i.e. its affinity for the test reagents). Furthermore, different methods exhibit such interferences to a greater or lesser extent (120,154). Because autoantibody interference is difficult for the laboratory to detect proactively, it is the physician who should first suspect interference from an unexpected discordance between the clinical presentation of the patient and the test result(s) (249,250).
TSH (THYROID STIMULATING HORMONE) MEASUREMENT
Over the last five decades the dramatic improvements in TSH assay sensitivity and specificity have revolutionized thyroid testing and firmly established TSH as the first-line test for ambulatory patients who are not receiving drugs known to alter TSH secretion (10,13,251). Serum TSH has become the therapeutic target for levothyroxine (L-T4) replacement therapy for hypothyroidism (52) and suppression therapy for differentiated thyroid cancer (DTC) (57,252,253). The diagnostic superiority of TSH versus FT4 measurement arises from the inverse, predominantly log/linear, TSH/FT4 relationship, that is modified to some extent by factors such as age, sex, active smoking, and TPOAb status (8,10,13,143,144,254,255).
TSH Assays
TSH assay "quality" has historically been defined by clinical sensitivity – the ability to discriminate between hyperthyroid and euthyroid TSH values (8,10,13,256). The first generation of RIA methods had a detection limit approximating 1.0 mIU/L (1,3,257) that limited their clinical utility to diagnosing primary hyperthyroidism (258) and necessitated the use of TRH stimulation to diagnose hyperthyroidism, characterized by an absent TRH-stimulated TSH response (259-261). With the advent of immunometric assay (IMA) methodology that uses a combination of poly- and/or monoclonal antibodies targeting different TSH epitopes in a "sandwich" format (262-264), a ten-fold improvement in TSH assay sensitivity (~ 0.1 mIU/L) was achieved when using isotopic (I125) signals (265). This level of sensitivity facilitated the determination of the lower TSH reference limit (0.3-0.4 mIU/L) and the detection of overt hyperthyroidism without the need for TRH stimulation (266,267) but was still insufficient for distinguishing between differing degrees of hyperthyroidism (i.e. subclinical versus overt) (268). Assay sensitization continued until a third generation of TSH IMAs was developed by employing non-isotopic signals that could achieve a sensitivity of 0.01 mIU/L (8,251,267). Initially different non-isotopic signals were used that gave rise to a lexicon of terminology to distinguish between assays: immunoenzymometric assays (IEMA) used enzyme signals; immunofluorometric assays (IFMA) used fluorophors as signals, immunochemiluminometric assays (ICMA) used chemiluminescent molecules as signals, and immunobioluminometric assays (IBMA) used bioluminescent signal molecules (112,267). Current TSH methods are mostly automated ICMAs that achieve third generation functional sensitivity (FS = ≤0.01 mIU/L) - a FS level that has now become the standard of care (10,13,269).
FUNCTIONAL SENSITIVITY (FS) = THE LOWEST REPORTABLE ASSAY LIMIT
During the period of active TSH assay sensitization, different non-isotopic IMAs made competing claims for sensitivity. Methods were described as: "sensitive", "highly sensitive", "ultrasensitive", or "supersensitive" - marketing terms that had no scientific definition. This confusion led to a debate concerning what was the most clinically relevant parameter to use to determine the lowest reliable reportable TSH value for clinical practice (10,251,267). Functional sensitivity (FS) became defined as the lowest analyte concentration measured with 20 percent coefficient of variation (10) established over a clinically relevant timespan (6-8 weeks for TSH). FS is now recognized as the parameter that best represents the between-run precision for measuring low analyte concentrations in clinical practice (10,270,271). FS is used to define the lower reportable limit for not only TSH but also Tg and TgAb, as well as other non-thyroid assays for which analytic sensitivity is critical (10). FS protocols recognize that immunoassays tend to be matrix-sensitive and specify that precision be determined in human sera rather than a quality control material that uses an artificial protein matrix (71,72,272). The timespan used for determining precision is also analyte-specific and should reflect the frequency of testing employed in clinical practice - 6 to 8 weeks for TSH, but 6 to 12 months for the Tg and TgAb - assays that are used as tumor markers for monitoring DTC. An optimal timescale is important, because low-end precision erodes over time due to a myriad of variables including reagent lot-to-lot variability (71). Note that the FS parameter is more stringent than other biochemical sensitivity parameters such as limit of detection (LOD - a within-run parameter) and limit of quantitation (LOQ - a between-run parameter without stipulations regarding the matrix and the timespan used for determining precision (72,271,273). A ten-fold difference in FS has been used to define each generation of increasingly more sensitive methods (17,251,271,274). Thus, TSH RIA methods with FS approximating 1.0 mIU/L were designated "first generation", the TSH immunoradiometric (IRMA) methods that had a functional sensitivity approximating 0.1 mIU/L were designated " second generation", and current TSH ICMAs with FS approximating 0.01 mIU/L are now designated "third generation" assays (267,270,275).
TSH BIOLOGIC VARIABILITY
TSH is a heterogeneous glycoprotein (276-278), and TRH-mediated changes in TSH glycosylation and thus detection by IMA methodology (279,280) have the potential to influence immunoactivity (277,281). Alterations in TSH glycosylation can occur in a number of pathophysiologic circumstances (278,282). Seasonal variability in TSH has been shown with 10% higher TSH levels in the winter than in the summer months (283). However, FT4 and FT3 levels show no such seasonal variability (283). The demonstration that harmonization of TSH methods successfully minimizes between-method differences (69), suggesting that under normal conditions current TSH IMAs appear to be "glycosylation blind" and detect different TSH glycoforms in an equimolar fashion (277,278). However, future studies need to include sera from conditions where TRH dysregulation may lead to abnormal TSH glycosylation and bioactivity, such as pituitary dysfunction, NTI, and aging (278,280,281,284-286).
TSH intra-individual variability is relatively narrow (20-25 percent) in both non-pregnant and pregnant subjects, as compared with between-person variability (13,287,288). In fact, the serum TSH of euthyroid volunteers was found to vary only ~0.5 mIU/L when tested every month over a span of one year (287). Twin studies suggest that there are genetic factors that determine hypothalamic-pituitary-thyroid setpoints (289-291). These studies report that the inheritable contribution to the serum TSH level approximates 65 percent (290,291). This genetic influence appears in part to involve single nucleotide polymorphisms in thyroid hormone pathway genes such as the phosphodiesterase gene (PDE8B) (292), polymorphisms causing gain (293) or loss of function TSH receptors (294), and the type II deiodinase enzyme polymorphisms (293). Undoubtedly, such polymorphisms account for some of the euthyroid outliers that skew TSH reference range calculations (295). The narrow TSH within-person variability and low (< 0.6) index of individuality (IoI) (287,288) limits the clinical utility of using the TSH population-based reference range to detect thyroid dysfunction in an individual patient (288,296-298). When evaluating patients with marginally (confirmed) low (0.1–0.4 mIU/L) or high (4–10 mIU/L) TSH abnormalities, it is more important to consider the degree of TSH abnormality relative to patient-specific risk factors for cardiovascular disease rather than the degree of the abnormality relative to the TSH reference range (13,52,299).
TSH REFERENCE RANGES
As with the thyroid hormone tests, the significant biases between different TSH methods (Figure 7) prevent establishing universal population or trimester-specific reference ranges that would apply across methods (13,170). These method biases also impact the detection of subclinical hypothyroidism (299,300). Since TSH is a complex glycoprotein, no reference measurement procedure (RMP) is available, or will likely be feasible in the future (13), given the current lack of commutability between the pituitary TSH reference preparations and patient specimens (33). A harmonization approach (31,301), whereby methods are recalibrated to the "all method mean", has been shown to have the potential to effectively eliminate current between-method TSH differences that are most pronounced at pathophysiologic levels (29,302). Better harmonization may also be possible using a reference panel of serum specimens (33). The IFCC is actively working with the IVD industry to encourage manufacturers to harmonize their methods. A reduction of between-method variability could eliminate the need to establish method-specific TSH reference ranges - a practice that is costly and inconvenient given the large numbers of rigorously screened participants that are necessary to establish reliable 2.5th to 97.5th percentiles for a population (87,303). However, even after harmonization minimizes inter-method differences, it remains to be determined to what extent universal ranges would be impacted by other factors such as age (254,304), ethnicity (254), and iodine intake (305). It may be that a reference range established in one geographic location may not be representative of a different locale or population. The harmonization of TSH methods would be advantageous for consolidating data from different studies and establishing universal reference limits (13).
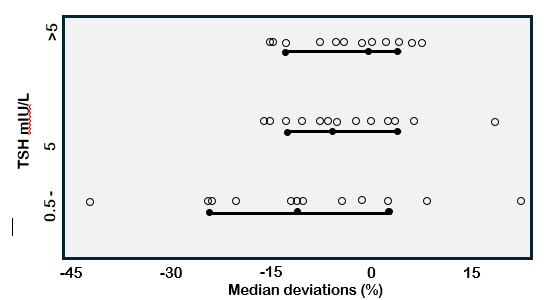
Figure 7. TSH Between-Method Variability. Figure shows deviations in TSH measurements made in the low (<0.5), medium (0.5-5.0), and high (>5) mIU/L range using 14 different immunoassays. Data is expressed as deviations from the trimmed all method mean (88).
TSH POPULATION REFERENCE RANGE
The log/linear TSH/FT4 relationship (8,10,143,144,255) dictates that TSH will be the first abnormality to appear as mild (subclinical) as hypo- or hyperthyroidism develops. It follows that the setting of the TSH reference limits critically influences the frequency of diagnosing subclinical thyroid disease (50,53). It is recommended that “TSH reference intervals should be established from the 95 percent confidence limits of the log-transformed values of at least 120 rigorously screened normal euthyroid volunteers who have: (a) no detectable thyroid autoantibodies, TPOAb or TgAb; (b) no personal or family history of thyroid dysfunction; (c) no visible or palpable goiter and, (d) who are taking no medications (except estrogen)” (10,303).
Multiple factors influence population TSH reference limits, especially the upper (97.5th percentile) limit. Different methods report different ranges for the same population resulting from between-methods biases (Figure 7) (13). A key factor affecting the upper limit is the stringency used for eliminating individuals with thyroid autoimmunity from the population (306-308). Other factors relate to population demographics such as sex (254), ethnicity (254,309,310), iodine intake (311,312), BMI (313,314), and smoking status (311,315). The relationship between TSH and age is complex with most studies in iodine sufficient populations reporting an increase in the TSH upper limit with age (143,254,308,309,316). This has led to the suggestion that age-and sex-specific TSH reference limits be used (50,316). Conflicting data on this issue could merely represent population differences with an increasing prevalence of thyroid autoimmunity in iodine-sufficient populations (254,317). Whereas in iodine deficient populations, increasing autonomy of nodular goiter can result in decreased TSH with aging (318). Some studies have reported that a mild TSH elevation in elderly individuals may convey a survival benefit (319), whereas other studies dispute this (320). However, TSH is a labile hormone, and studies cannot assume that a TSH abnormality found in a single determination is representative of thyroid status in the long-term (321).
PEDIATRIC TSH REFERENCE RANGES
The adult TSH population reference range does not apply to neonates or children. Serum TSH values are generally higher in neonates and then gradually decline until the adult range is reached after puberty (178,179,322,323). This necessitates using age-specific TSH reference ranges for diagnosing thyroid dysfunction in different pediatric age groups.
SUBCLINICAL THYROID DYSFUNCTION
Subclinical Hyperthyroidism (SCHY) is defined as a low (<2.5th percentile) but detectable TSH (0.01 - 0.3 mIU/L range) without a FT4 abnormality. SCHY seems relatively independent of the method used (324-326). Endogenous SCHY prevalence is low (0.7 %) in iodine-sufficient populations (254) but may increase as an iatrogenic consequence of L-T4 replacement therapy (327-330). SCHY is a risk factor for osteoporosis and increased fracture risk (331) as well as atrial fibrillation and cardiovascular disease (325,332-334), especially in older patients.
Subclinical Hypothyroidism (SCHO) is defined as a TSH above the upper (>97.5th percentile) TSH reference limit without a FT4 abnormality (50,300,308,335). However, the setting of the TSH upper limit remains controversial, thus the prevalence of SCHO is highly variable - 4 to 8.5 percent rising to 15 percent in older populations (254,299,307,335). In most cases, SCHO is associated with TPOAb positivity, indicative of an autoimmune etiology (307). The clinical consequences of SCHO relate to the degree of TSH elevation (336,337). Most guidelines recommend L-T4 treatment of SCHO when TSH is above 10 mIU/L (49,50), but below 10 mIU/L L-T4 treatment is usually based on patient-specific risk factors (50). There is active debate concerning the efficacy of treating SCHO to prevent progression (338-340), or improve renal (341), cardiovascular (333,336,342-346), or lipid (347,348) abnormalities that can be associated with SCHO.
THYROID DYSFUNCTION IN PREGNANCY
Overt hypo- or hyperthyroidism is associated with both maternal and fetal complications (349-352). However, the impact of maternal subclinical thyroid dysfunction remains controversial (51), although no maternal or fetal complications appear associated with subclinical hyperthyroidism during pregnancy (349,353). First trimester "gestational hyperthyroidism" is typically transient and hCG-related (354). In contrast, short-term and long-term outcome studies of maternal subclinical hypothyroidism (51,355) are complicated by heterogeneity among studies arising from a myriad of factors influencing TSH cutoffs, such as gestational stage, TSH method used, maternal TPOAb status, and current and pre-pregnancy iodine intake (160,172). Using gestational age-specific reference intervals the frequency of SCHO in first trimester pregnancy approximates 2-5 percent (355,356). Studies have found that subclinical hypothyroidism is associated with increased frequency of maternal and fetal complications, especially when TPOAb is positive (51,160,349,357-362). Maternal complications have included miscarriage (358), preeclampsia (363,364), placental abruption (350), preterm delivery (349,358,365,366), and post-partum thyroiditis (359). Fetal complications have included intrauterine growth retardation and low birth weight (350,353) and possible impaired neuropsychological development (367,368). It remains controversial whether L-T4 treatment of SCHO in early gestation decreases the risk of complications (358,362,369).
Trimester-Specific TSH Reference Ranges. As with non-pregnant patients, TSH is the first-line test used for assessing thyroid status during pregnancy when gestation-related TSH changes occur (47,51,76,158,355). Currently, method specific TSH reference ranges are needed for each trimester because of between-method variability (Figure 8). In the first trimester, there is a transient rise in FT4 caused by high hCG concentrations stimulating the TSH receptor - because hCG shares some homology with TSH (370-372). The degree of TSH suppression is inversely related to the hCG concentration and can be quite profound in patients with hyperemesis who have an especially high hCG (165,370,372-374). As gestation progresses, TSH tends to return towards pre-pregnancy levels (165). Recent studies from different geographic areas with diverse iodine intakes using different TSH methods have reported higher trimester-specific TSH upper limits than recommended by previous guidelines (51,159,164,165, 355, 375). In response, the American Thyroid Association have revised their pregnancy guidelines (47,48) to replace trimester-specific reference limits by a universal upper TSH limit of 4.0 mIU/L, when TPOAb is negative and no local reference range data is available (376). However, at this time between-method biases (Figure 7) clearly preclude proposing universal TSH cut offs that would apply to all methods and all populations including pregnant patients (69,87,164,165). IVD manufacturers are being encouraged to harmonize their TSH methods so that universal reference limits can be established for pregnancy (69,87). Requiring each institution to establish their own trimester-specific reference ranges is impractical, given the costs, logistics and ethical considerations involved in recruiting the more than 400 disease-free pregnant women that would be needed to represent each trimester (158). Even after methods are re-standardized (FT4) or harmonized (TSH), trimester-specific reference ranges would still be influenced by differences in ethnicity and iodine intake, especially the pre-pregnancy iodine intake that influences thyroidal iodine stores (172). In addition, since the TSH upper limit is skewed by the inclusion of individuals with thyroid autoimmunity, reliable method-specific TPOAb cutoffs need to be established (165,372,377).
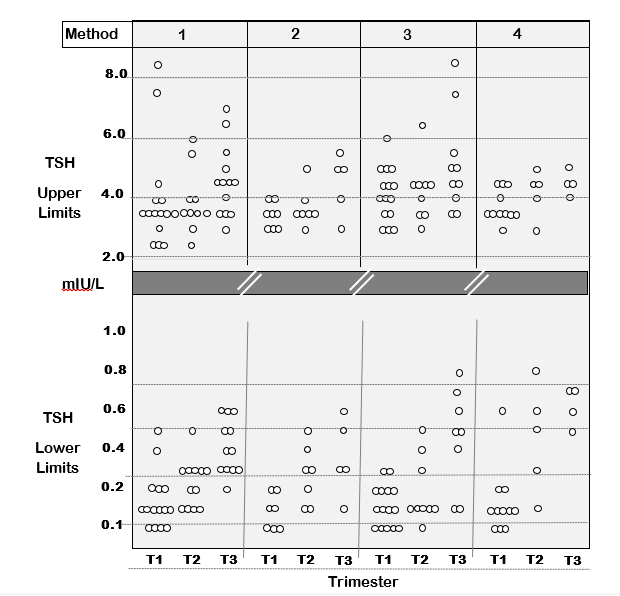
Figure 8. Between-Method TSH Variability Impacts Thyroid Testing in Pregnancy. The figure is a summary of 43 published studies showing the upper and lower TSH reference limits (2.5–97.5 %) measured in each trimester of pregnancy by four different methods – Abbott (1), Beckman (2), Roche (3), and Siemens (4). The data shows the expected trend for a lower TSH in the first trimester, resulting from thyroidal human chorionic gonadotropin (HCG) stimulation of thyroxine, which is maximal in the first trimester. The data is re- drawn with permission from reference 156.
Clinical Utility of TSH Measurement
AMBULATORY PATIENTS
In the outpatient setting the reliability of TSH testing is not usually influenced by the time of day of the blood draw, because the diurnal TSH peak occurs between midnight and 0400 (378-381). However, seasonal changes in TSH have been shown, with TSH approximately 10 % higher in winter than in summer (283). Third generation TSH assays (FS ~0.01 mIU/L) have now become the standard of care because they can reliably detect the full spectrum of thyroid dysfunction from overt hyperthyroidism to overt hypothyroidism, provided that hypothalamic-pituitary function is intact, and thyroid status stable (10,49,382,383). TSH is also used for optimizing L-T4 therapy - a drug with a narrow therapeutic range (49,384). Because TSH secretion is slow to respond to changes in thyroxine status there is no need to withhold the L-T4 dose on the day of the blood test (10,384). In addition, in differentiated thyroid cancer (DTC) patients, targeting the degree of TSH suppression relative to recurrence risk plays a critical role in management (57,385,386).
HOSPITALIZED PATIENTS WITH NONTHYROIDAL ILLNESSES (NTI)
Non-thyroidal illness, sometimes called the "sick euthyroid syndrome" is associated with alterations in hypothalamic/pituitary function and thyroid hormone peripheral metabolism, often exacerbated by drug influences (104,181,186,387-389). Routine thyroid testing in the hospital setting is not recommended because thyroid test abnormalities are frequently seen in sick euthyroid patients (Figure 6) (388-391). TSH also usually remains within normal limits or may become somewhat depressed in the early phase, especially in response to drug therapies such as dopamine or glucocorticoid (104,110,181). During the recovery phase, TSH frequently rebounds above the reference range (184). High TSH may also be seen associated with psychiatric illness (392). It is important to distinguish the generally mild, transient TSH alterations typical of NTI from the more profound and persistent TSH changes associated with hyper- or hypothyroidism (10,183,185,390).
Causes of Misleading TSH Measurements
A diagnostically misleading TSH can result from biological factors or interferants in the serum such as drugs, heterophile antibodies (i.e. HAMA), or endogenous TSH autoantibodies (91,195,197,378,393). In most cases such interferences cause a falsely high TSH.
BIOLOGIC FACTORS CAUSING MISLEADING TSH
Unstable Thyroid Function
TSH can be misleading when there is unstable thyroid status - such as in the early phase of treating hypo- or hyperthyroidism or non-compliance with L-T4 therapy - when there is a lag in the resetting of pituitary TSH to reflect a new thyroid status (394). During such periods of instability TSH will be misleading and FT4 will be the more diagnostically reliable test.
Pituitary/Hypothalamic Dysfunction
Pituitary dysfunction is rare in ambulatory patients (395). TSH measurement is unreliable in cases of both central hypothyroidism and central hyperthyroidism (285,395-397).
Central hypothyroidism (CH) is rare, 1/1000 less prevalent than primary hypothyroidism, 1/160,000 detected by neonatal screening) (395). CH can arise from disease at either the pituitary or hypothalamic level, or both (395). A major limitation of using a TSH-centered screening strategy is that current TSH tests will miss a diagnosis of CH because TSH IMAs are “glycosylation blind” and detect the abnormally glycosylated biologically inactive TSH as “normal” TSH, despite clinical hypothyroidism (276,285,396). This limitation necessitates that the clinical diagnosis of CH be confirmed biochemically as a low FT4/normal-low TSH discordance. and that L-T4 replacement therapy for CH be optimized using the serum FT4 not TSH. It should be noted that in the absence of clinical suspicion, investigations for pituitary dysfunction should only be initiated after ruling-out technical interference.
TSH secreting pituitary adenomas are characterized by a non-suppressed TSH associated with high thyroid hormone levels and clinical hyperthyroidism (397,398). Since this is a rare type of pituitary adenoma (0.7 %), technical interferences such as heterophile antibody (HAb) or TSH autoantibodies (macro TSH) should be excluded before initiating inconvenient and unnecessary pituitary imaging or dynamic diagnostic testing such as T3 suppression or TRH stimulation. This clinical/biochemical discordance reflects the TSH isoforms with enhanced biologic activity secreted by the adenoma. As with CH, current TSH IMA methods cannot distinguish these abnormal isoforms from normal TSH. Failure to diagnose the pituitary as the cause of the hyperthyroidism can lead to inappropriate thyroid ablation. The treatment of choice is surgery but in cases of surgical failure somatostatin analog treatment has been found effective (398). Note that the biochemical profile (high thyroid hormones and non-suppressed TSH) resembles that seen with thyroid hormone resistance syndromes (399,400) or interference from thyroid autoantibodies (120).
Resistance to Thyroid Hormone (RTH)
Resistance to thyroid hormone is caused by mutations in the THRB gene encoding the thyroid hormone receptor Band is biochemically characterized by high thyroid hormone (FT4 +/- T3) levels and a non-suppressed, sometimes slightly elevated TSH (402,403). Tissues expressing primarily the thyroid hormone receptor B are hypothyroid (e.g. the liver), whereas organs with a predominant expression of thyroid receptor A (e.g. the heart) display alterations consistent with thyroid hormone excess (400,401). Early cases of thyroid hormone resistance were shown to result from mutations in the thyroid hormone receptor B (400). More recently the syndromes with decreased sensitivity to thyroid hormones have been broadened to include mutations in thyroid hormone transporters (e.g. MCT8), the metabolism of thyroid hormone (e.g. SBP2), and resistance mediated by mutations in thyroid receptor A (401) (for detailed discussion see the Endotext chapter entitled “Impaired Sensitivity to Thyroid Hormone: Defects of Transport, Metabolism and Action”). These insensitivity and resistance syndromes display a spectrum of clinical and biochemical profiles and can now be identified by genetic testing.
Activating or Inactivating TSH Receptor Mutations
Non-autoimmune hyperthyroidism resulting from an activating mutation of the TSH receptor (TSHR) is rare (293,402). A spectrum of loss-of-function TSHR mutations (TSH resistance) causing clinical and subclinical hypothyroidism despite high thyroid hormone levels, have also been described (295,400,403,404). Because TSHR mutations are a rare cause of TSH/FT4 discordances, technical interferences should first be excluded before considering a TSHR mutation as the cause of these discordant biochemical profiles.
TECHNICAL FACTORS CAUSING MISLEADING TSH
Non-Analyte Specific Interferences
Heterophile Antibodies (HAbs) such as Human Anti Mouse Antibody (HAMA) can cause falsely high TSH IMA tests (200,220,241,405,406) and interfere with neonatal TSH screening (407). Since the HAb in some patient's sera interfere strongly with some manufacturers tests but appear inert in others (200), re-measurement using a different manufacturers assay should be the first test to identify interference. A fall in TSH in response to a blocker-tube treatment (43) is typically used to confirm HAb interference.
Anti-Reagent Antibody Interferences. As discussed for free hormone tests, some patients have antibodies that target test reagents such as rhuthenium and cause interference with TSH tests. (408). It should be noted that the anti-rhuthenium antibodies of different patients may affect different analytes to differing degrees (230,409,410).
Biotin Interferences. Tests employing streptavidin or biotin reagents are prone to interferences from antibodies targeting either streptavidin (231) or biotin (233). Alternatively, high dose biotin ingestion has been known to produce interference in an analyte-specific, platform-specific manner (241,411). The popularity of biotin therapy is now prompting assay manufacturers to reformulate their tests to remove biotin interference (237,412).
Analyte-Specific Interferences
Analyte-specific interferences typically result from autoantibodies targeting the analyte. Depending on the analyte and test formulation, autoantibody interferences most commonly cause falsely high test results. It should be noted that transplacental passage of both heterophile antibodies or anti-analyte autoantibodies (i.e. TSHAb or T4Ab) have the potential to interfere with neonatal screening tests (245-247,413). Patients with autoantibodies targeting both TSH and prolactin (PRL) have been described (414).
TSH Autoantibodies (Macro TSH). Analytically suspicious TSH measurements are not uncommon (205,238,239,244,415) and have been reported in up to five percent of specimens subjected to rigorous screening (405). There have been many reports of TSHAb, often referred to as "macro TSH” causing spuriously high TSH results in a range of different methods used for both adult (238,416) and neonatal screening (244,415). The prevalence of TSHAb approximates 0.8 percent but can be as high as 1.6 percent in patients with subclinical hypothyroidism (238). The most convenient test for TSHAb is to show a lowering of TSH in response to a polyethylene glycol (PEG) precipitation of immunoglobulins (415-417). Alternatively, column chromatography can show TSH immunoactivity in a high molecular weight peak representing a bioinactive TSH-immunglobulin complex (415,416).
TSH Variants. TSH variants are a rare cause of interference (403). Nine different TSH beta variants have been identified to date (286). These mutant TSH molecules may have altered immunoactivity and be detected by some TSH IMA methods but not others (403). The bioactivity of these TSH mutants is variable and can range from normal to bio-inert (286,403), resulting in discordances between the TSH concentration and clinical status (403) and/or a discordant TSH/FT4 relationship (286). These TSH genetic variants are one of the causes of central congenital hypothyroidism (418,419).
THYROID SPECIFIC AUTOANTIBODIES (TRAb, TPOAb and TgAb)
Tests for antibodies targeting thyroid-specific antigens such as thyroid peroxidase (TPO), thyroglobulin (Tg) and TSH receptors (TSHR) are used as markers for autoimmune thyroid conditions (420-422). Over the last four decades, thyroid antibody test methodologies have evolved from semi-quantitative agglutination, complement fixation techniques and whole animal bioassays to specific ligand assays using recombinant antigens or cell culture systems transfected with the human TSH receptor (20,420). Unfortunately, the diagnostic and prognostic value of these tests has been hampered by methodologic differences as well as difficulties with assay standardization (423,424). Although most thyroid autoantibody testing is currently made on automated immunoassay platforms, methods vary in sensitivity, specificity, and the numeric values they report because of standardization issues (45,377,425). Thyroid autoantibody testing can be useful for diagnosing or monitoring treatment for several clinical conditions, although these tests should be selectively employed as adjunctive tests to other diagnostic testing procedures.
TSH Receptor Autoantibodies (TRAb)
The TSH receptor (TSHR) serves as a major autoantigen (19,422,426-428). Thyroid gland stimulation occurs when TSH binds to the TSHR on thyrocyte plasma membranes and activates the cAMP and phospholipase C signaling pathways (427). The TSH receptor belongs to the G protein-coupled class of transmembrane receptors. It undergoes complex posttranslational processing in which the ectodomain of the receptor is cleaved to release a subunit into the circulation (426). The TSH-like thyroid stimulator found uniquely in the serum of Graves’ disease patients was first described using a guinea pig bioassay system in 1956 (429). Later, a mouse thyroid bioassay system was used to show this serum factor displayed a prolonged stimulatory effect as compared to TSH and hence was termed to be a “long-acting thyroid stimulator” or LATS (430,431). Much later, the LATS factor was recognized not to be a TSH-like protein but an antibody capable of stimulating the TSH receptor that was the cause of Graves’ hyperthyroidism (432). TSH receptor antibodies (TRAb) have also become implicated in the pathogenesis of Graves’ ophthalmopathy (432-436). TRAbs are heterogeneous (polyclonal) and fall into two general classes both of which can be associated with autoimmune thyroid disorders – (a) thyroid stimulating autoantibodies (TSAb) that mimic the actions of TSH and cause Graves’ hyperthyroidism and (b), blocking antibodies (TBAb) that block TSH binding to its receptor and can cause hypothyroidism (19,20,420,427,432,437-440). TSH, TSAb and TBAb appear to bind to different sites on the TSH receptor ectoderm with similar affinities and often overlapping epitope specificities (441). In some cases of Graves’ hyperthyroidism, TBAb have been detected in association with TSAb (442,443) and the dominance of one over the other can change over time in response to treatment (444,445). Because both TSAb and TBAb can be present in the same patient, the relative concentrations and receptor binding characteristics of these two classes of TRAb can influence the severity of Graves’ hyperthyroidism and the response to antithyroid drug therapy or pregnancy (426,442,446-448). For completeness, it should also be mentioned that a third class of “neutral” TRAb has also been described, of which the functional significance has yet to be determined (432,438,448,449).
Two different methodologic approaches have been used to quantify TSH receptor antibodies (425,437,450,451): (a) TSH receptor antibody tests (TRAb assays) also called TSH Binding Inhibition Immunoglobulin (TBII) assays, and (b) Bioassays that use whole cells transfected with human or chimeric TSH receptors that produce a biologic response (cAMP or bioreporter gene) when TSAb or TBAb are present in a serum specimen. In recent years automated immunometric assays using recombinant human TSHR constructs have been shown to have high sensitivity for reporting positive results in Graves' disease sera (18,425). However, assay sensitivity varies among current receptor versus bioassay methods (452).
TSH RECEPTOR (TRAb)/TSH BINDING INHIBITORY IMMUNOGLOBULIN (TBII)
TRAb methods detect serum immunoglobulins that bind TSHR but do not functionally discriminate stimulating from blocking antibodies (453). TRAb methods are based on standard competitive or noncompetitive principles. The first generation of methods were liquid-based whereby immunoglobulins in the serum inhibited the binding of 125I-labeled TSH or enzyme-labeled TSH to a TSH receptor preparation (451). These methods used TSH receptors of human, guinea pig, or porcine origin (454). After 1990, a second generation of both isotopic and non-isotopic methods were developed that used and immobilized porcine or recombinant human TSH receptors (451,455). These second-generation methods were shown to have significantly more sensitivity for detecting Graves' thyroid stimulating immunoglobulins than first generation tests (425). In 2003 a third generation of non-isotopic methods were developed that were based on serum immunoglobulins competing for immobilized TSHR preparation (recombinant human or porcine TSHR) with a monoclonal antibody (M22) (420,425,451,455-457). Third generation assays have also shown a good correlation and comparable overall diagnostic sensitivity with bioassay methods (425,442,458). Current third generation TRAb tests have now been automated on several immunoassay platforms (425). However, between-method variability remains high and between assay precision is often suboptimal (CVs > 10 %) despite calibration using the same International Reference Preparation (08/204) (423,459). This fact makes it difficult to compare values using different methods and indicates that further efforts focused on additional assay improvements are needed (420,423,455).
Over the last ten years automated IMA methods have dramatically lowered the cost and increased the availability of TRAb testing (18,428,452). Automated TRAb IMAs are not functional tests and do not distinguish between stimulating and blocking TRAbs (455), however, this distinction is usually unnecessary, since it is evident from clinical evidence of hyper- or hypothyroid features. Also, both TSHR stimulating and blocking antibodies may be detected simultaneously in the same patient and cause diagnostic confusion (460). Because the sensitivity and specificity of current third generation TRAb tests is over 98 percent, TRAb testing can be useful for determining the etiology of hyperthyroidism (425,428), as an independent risk factor for Graves’ ophthalmopathy (435,436,440), and may be useful for monitoring responses to therapy (76,425). TRAb measured prior to radioiodine therapy for Graves' hyperthyroidism can also help predict the risk for exacerbating ophthalmopathy (433,436,461). There is conflicting data concerning the value of using TRAb to predict the response to antithyroid drug treatment or the risk of relapse (443,458,462,463). An important application of TRAb testing is to detect high TRAb concentrations in pregnant patients with a history of autoimmune thyroid disease or active or previously treated Graves’ hyperthyroidism, in whom transplacental passage of stimulating or blocking TRAb can cause neonatal hyper- or hypothyroidism, respectively (76,352,425,437,451,464-466). Because the expression of thyroid dysfunction may be different in the mother and infant, automated IMA methods have the advantage of being able to detect both stimulating and blocking antibodies (467). It is currently recommended that TRAb be measured in the first trimester in all pregnant patients with active Graves’ hyperthyroidism or who have received prior ablative (radioiodine or surgery) therapy for Graves’ disease in whom TRAb can remain high even after patients have been rendered hypothyroid and are being maintained on L-T4 replacement therapy (47,48). When TRAb is high in the first trimester additional TRAb testing is recommended at 18-22 and 30-34 weeks (47,48,76,420,442,468).
BIOASSAY METHODS (TSAb/TBAb)
The first TSH receptor assays used surgical human thyroid specimens, mouse, or guinea pig thyroid cells, or rat FRTL-5 cell lines to detect TSH receptor antibodies. These methods typically required pre-extraction of immunoglobulins from the serum specimen (429,437,439,469,470). Later, TRAb bioassays used cells with endogenously expressed or stably transfected human TSH receptors and unextracted serum specimens (471-473). Current TRAb bioassays are functional assays that use intact (typically CHO) cells transfected with human or chimeric TSH receptors, which when exposed to serum containing TSH receptor antibodies use cAMP or a reporter gene (luciferase) as a biological marker for any stimulating or blocking activity in a serum (425,451,463). Bioassays are more technically demanding than the more commonly used receptor assays because they use viable cells. However, these functional assays can be modified to detect TBAb that may coexist with TSAb in the same sera and make interpretation difficult (451). The most recent development is for second generation assays to use a chimeric human/rat LH TSHR to effectively eliminate the influence of blocking antibodies. This new approach has shown excellent sensitivity and specificity for diagnosing Graves' hyperthyroidism and clinical utility for monitoring the effects of anti-thyroid drug therapy (463).
Thyroid Peroxidase Autoantibodies (TPOAb)
TPO is a large, dimeric, membrane-associated, globular glycoprotein that is expressed on the apical surface of thyrocytes. TPO autoantibodies (TPOAb) found in sera typically have high affinities for an immunodominant region of the intact TPO molecule. When present, these autoantibodies vary in titer and IgG subclass and display complement-fixing properties (474). Studies have shown that epitope fingerprints are genetically conserved suggesting a possible functional importance (475). However, it is still unclear whether the TPOAb epitope profile correlates with the presence of, or potential for, the development of thyroid dysfunction (474-477). TPOAb antibodies were initially detected as antibodies against thyroid microsomes (antimicrosomal antibodies, AMA) using semi-quantitative complement fixation and tanned erythrocyte hemaagglutination techniques (478). Studies have identified the principal antigen in AMA tests as the thyroid peroxidase (TPO) enzyme, a 100 kD glycosylated protein present in thyroid microsomes. Manual agglutination tests have now been replaced by more specific, automated TPOAb immunoassay or immunometric assay methods that use purified or recombinant TPO (10,420,479-482). There is considerable inter-method variability of current TPOAb assays (correlation coefficients 0.65 and 0.87), despite calibration against the same International Reference Preparation (MRC 66/387) (420,479,480,482). It appears that both the methodologic principles of the test and the purity of the TPO reagent used may influence the sensitivity, specificity, and reference range of the method (420,479). The variability in sensitivity limits and the reference ranges of different methods has led to different interpretations regarding the normalcy of having a detectable TPOAb (377,420,424,482).
TPOAb CLINICAL SIGNIFICANCE
Estimates of TPOAb prevalence depend on the sensitivity and specificity of the method employed (377,424,482). In addition, ethnic and/or geographic factors (such as iodine intake) influence the TPOAb prevalence in population studies (317). For example, TPOAb prevalence is significantly higher (~11 percent) in countries like the United States and Japan where dietary iodine is sufficient, as compared with iodine deficient areas in Europe (~ 6 percent) (254,483). The prevalence of TPOAb is higher in women of all age groups and ethnicities, presumably reflecting the higher propensity for autoimmunity as compared with men (254,483). Approximately 70-80 percent of patients with Graves' disease and virtually all patients with Hashimoto’s or post-partum thyroiditis have detected TPOAb (479,484). TPOAb has, in fact, been implicated as a cytotoxic agent in the destructive thyroiditic process (477,485,486).
TPOAb prevalence is also significantly higher in various non-thyroidal autoimmune disorders in which no apparent thyroid dysfunction is evident (487). Aging is associated with an increasing prevalence of TPOAb that parallels the increasing prevalence of both subclinical and clinical hypothyroidism (254). In fact, the NHANES III survey reported that TPOAb prevalence increases with age and approaches 15-20 percent in elderly females even in the iodine-sufficient United States (254). This same study found that the odds ratio for hypothyroidism was strongly associated with the presence of TPOAb but not TgAb, suggesting that primarily TPOAb contribute to the autoimmune etiology of hypothyroidism (254). Although the presence of TgAb alone did not appear to be associated with hypothyroidism or TSH elevations, the combination of TPOAb and TgAb versus TPOAb alone may be more pathologically significant (Figure 9), however further studies would be needed to confirm this (254,307,477). It is now apparent that the presence of TPOAb in apparently euthyroid individuals (TSH within reference range) appears to be a risk factor for future development of overt hypothyroidism that subsequently becomes evident at the rate of approximately two percent per year in such populations (474,488,489). Furthermore, TPO-positivity in pregnant women is a risk factor for preterm birth (490).
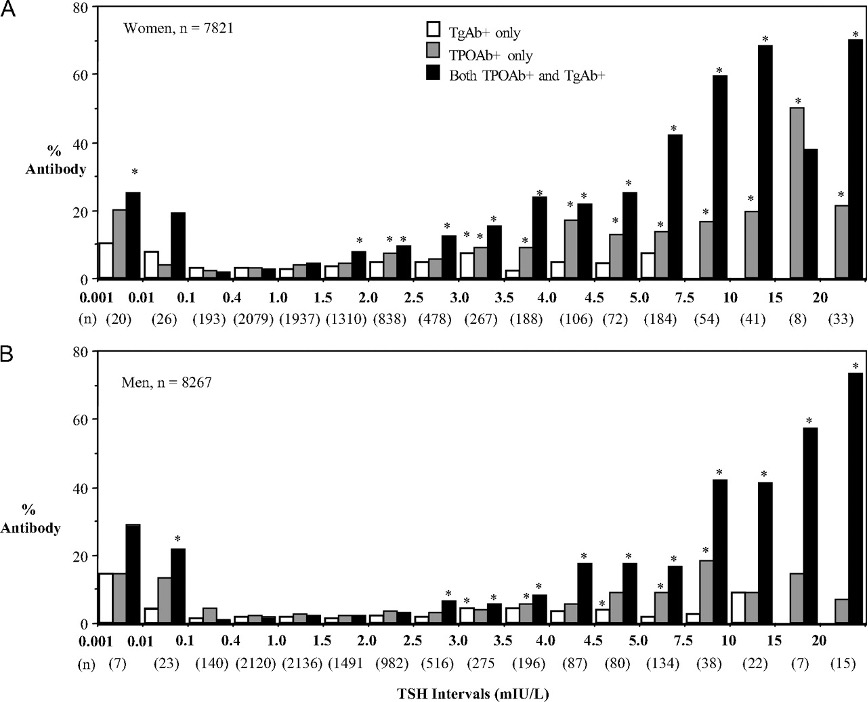
Figure 9. Prevalence of thyroid antibodies in women (A) and men (B). Abscissa TSH values correspond to the upper and lower limits of the intervals spanning each set of bars. Asterisks denote a significant difference in prevalence from the TSH range with lowest antibody prevalence, 0.1 and 1.5 mIU/liter for women and 0.1 and 2.0 mIU/liter for men from reference 307.
TPOAb measurement can serve as a useful prognostic indicator for future thyroid dysfunction (489,491). However, a hypoechoic ultrasound pattern can often be seen before the biochemical TPOAb abnormality appears (492). Further, some individuals with unequivocal TSH elevations, presumably resulting from autoimmune destructive disease of the thyroid, do not have TPOAb detected (307). Presumably, this paradoxical absence of TPOAb in some patients with elevated TSH likely reflects the suboptimal sensitivity and/or specificity of current TPOAb tests or a non-autoimmune cause of thyroid failure (i.e. atrophic thyroiditis) (254,307,482,493).
Although changes in autoantibody concentrations often occur with treatment, or reflect a change in disease activity, serial TPOAb measurements are not recommended for monitoring treatment for autoimmune thyroid diseases (49,479,494). This is not surprising since treatment of these disorders addresses the consequence (thyroid dysfunction) and not the cause (autoimmunity) of the disease. However, where it may have an important clinical application is to use the presence of serum TPOAb as a risk factor for developing thyroid dysfunction in patients receiving amiodarone, interferon-alpha, interleukin-2, or lithium therapies which all appear to act as triggers for initiating autoimmune thyroid dysfunction in susceptible (especially TPOAb-positive) individuals (10,110,495-500).
During pregnancy the presence of TPOAb has been linked to reproductive complications such as miscarriage, infertility, IVF failure, fetal death, pre-eclampsia, pre-term delivery, post-partum thyroiditis, and depression (47,76,484,501-508). However, whether this association represents cause or effect remains unresolved.
Thyroglobulin Autoantibodies (TgAb)
Thyroglobulin autoantibodies belong predominantly to the immunoglobulin G (IgG) class, are not complement fixing and are generally conformational (509). Tg autoantibodies were the first thyroid antibody to be detected in the serum of patients with autoimmune thyroid disorders using tanned red cell hemagglutination techniques (478). Subsequently, methodologies for detecting TgAb have evolved in parallel with those for TPOAb measurement, from semi-quantitative techniques to more sensitive ELISA and RIA methods and now to non-isotopic competitive or non-competitive immunoassays (45,420,482,510,511). Unfortunately, the between-method variability of TgAb assays is even greater than that of the TPOAb tests (Figure 10) (45,420,510-512). Additionally, high levels of thyroglobulin in the serum have the potential to influence TgAb measurements (511). Between-method variability is influenced by the purity and the epitope specificity of the Tg reagent, as well as the patient-specific epitope specificity of the TgAb secreted (513). As with TPOAb methods, TgAb tests have highly variable sensitivity limits and manufacturer-recommended cut-off values for "positivity", despite the use of the same International Reference Preparation (MRC 65/93) (Figure 10) (45,510-512,514). Whereas the FS limit is the recommended cutoff to define TgAb-positivity for DTC monitoring, the FS is typically much lower than the manufacturer-recommended cut-off for “positivity” (Figure 10) (10,45). This is because manufacturer-recommended cutoffs (MCO) are set for diagnosing thyroid autoimmunity and are too high to detect the low TgAb levels that can interfere with Tg measurements (515,516). Although there are reports that low levels of TgAb may be present in normal euthyroid individuals, it is unclear whether this represents assay noise due to matrix effects or "natural" antibodies (21). Further complicating this question are studies suggesting that there may be qualitative differences in TgAb epitope specificities expressed by normal individuals versus patients with either differentiated thyroid cancers (DTC) or autoimmune thyroid disorders (517). These differences in test sensitivity and specificity negatively impact the reliability of determining the TgAb status (positive versus negative) of specimens prior to Tg testing of DTC patients.
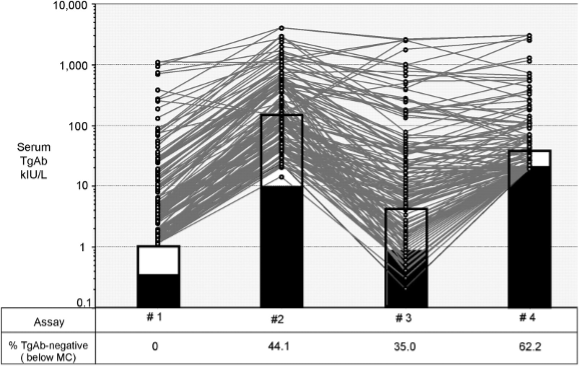
Figure 10. TgAb Measurements Made by Different Methods. Figure shows the relative TgAb concentrations reported for 143 DTC patient sera with evidence of TgAb interference with serum Tg measurements. Each serum was measured by four different methods each with a different manufacturer-recommended cutoff value for “TgAb positivity” (open bars) and with different experimentally determined (10) functional sensitivity limits (closed bars). From reference 45.
CLINICAL UTILITY OF TgAb TESTING
Tg autoantibodies (TgAb) are encountered in autoimmune thyroid conditions, usually in association with TPOAb (254,489,490). However, the NHANES III survey found that only three percent of subjects with no risk factors for thyroid disease had serum TgAb present without detectable TPOAb (Figure 9) (254,307). Furthermore, there was no association between the isolated presence of TgAb and TSH abnormalities in these subjects (254,307). This suggests that it is unnecessary to measure both TPOAb and TgAb for a routine evaluation for thyroid autoimmunity (307,420,489). However, when autoimmune thyroid disease is present, there is some evidence that assessing the combination of TPOAb and TgAb has greater diagnostic utility than the TPOAb measurement alone (Figure 9) (307,489,490,518). In pregnant women, both TPOAb and TgAb-positivity have been shown to be risk factors for preterm birth (490).
The role of TgAb for monitoring patients with DTC is two-fold: 1) to authenticate that a Tg measurement is not compromised by TgAb interference, and 2) as an independent surrogate tumor-marker (519,520). Immunoassay methods detect TgAb in approximately 25 percent of patients presenting with DTC, double the TgAb prevalence of the general population (45,254,521,522). In patients with thyroid nodules the presence of TgAb is a risk factor for lymph node metastases (520,523,524) and may be a useful marker for papillary thyroid cancer in cases of indeterminate cytology (523,525,526). The prevalence of TgAb is typically higher in patients with papillary versus follicular tumors (22,510,519,527-529). After TgAb-positive patients are rendered disease-free by surgery, TgAb concentrations typically progressively decline during the first few post-operative years and typically become undetectable after a median of three years of follow-up (22,530,531). In contrast, a rise in, or de novo appearance of, TgAb is often the first indication of tumor recurrence (14,22,531,532). In patients with persistent disease, serially determined TgAb concentrations may serve as an independent surrogate tumor marker for changes in tumor mass (Figure 11) (14,17,22,520,530,531,533-536). However, the use of the TgAb trend as a surrogate tumor marker necessitates that TgAb be measured by the same method in preferably the same laboratory, because of the large differences in the sensitivities and cut off values for “positivity” between different methods (Figure 10) (9,45,511,512,514,519,521).
THYROGLOBULIN (Tg)
Thyroglobulin plays a central role in a variety of pathophysiologic thyroid conditions, including acting as an autoantigen for thyroid autoimmunity (421,509,537). Serum Tg levels can serve as a marker for iodine status of a population (538-540) and genetic defects in Tg biosynthesis causing dyshormonogenesis can result in congenital hypothyroidism (10,541,542). Because Tg has a thyroid-tissue specific origin, a serum Tg measurement can be used to investigate the etiology of congenital hypothyroidism (athyreosis versus dyshormonogenesis) (543,544). Likewise, a paradoxically low serum Tg can be used to distinguish factitious hyperthyroidism from the high Tg expected with endogenous hyperthyroidism (14,545-547). However, the primary clinical use of Tg measurement is as a post-operative tumor-marker test used to monitor patients with follicular-derived (differentiated) thyroid cancer (DTC) (14,17,57,271,274,548-550).
Most Tg testing is currently by rapid, automated immunometric assays (IMA), most of which now have second generation functional sensitivity (FS≤ 0.1 µg/L) - a sensitivity level that obviates the need for recombinant human TSH (rhTSH) stimulation (57,274,551-554). TgAb interference, causes falsely low/undetectable serum Tg IMA tests and this is the major limitation of using IMA methodology since this direction of interference can mask disease (14,17,23,512,521,555,556). Currently, most laboratories first establish the TgAb status of the specimen (negative or positive) and restrict Tg-IMA testing to TgAb-negative sera, while reflexing TgAb-positive specimens to other methodologies believed less prone to TgAb interference from TgAb - RIA (14,274,512,521) or LC-MS/MS (14,24,43,555,557,558).
Technical Limitations of Tg Methods
Thyroglobulin measurement remains technically challenging. Five methodologic problems impair the clinical utility of this test: (a) suboptimal functional sensitivity; (b) between-method biases; (c) "hook" problems (some IMA methods) and interferences caused by (e) Heterophile antibodies (HAb) and/or (f) Tg autoantibodies (TgAb).
Tg ASSAY SENSITIVITY
As with TSH, assay functional sensitivity (FS) represents the lowest analyte concentration that can be measured in human serum with 20 percent CV, calculated from runs made over a clinically relevant timespan (6 -12 months for Tg) and using at least two different lots of reagents (10). These stipulations are necessary because assay precision erodes over time, especially during the long clinical interval (6-12 month) typically used when monitoring Tg as a tumor marker for DTC, during which time assay reagents and conditions can change (9,71,559). The use of FS as the assay sensitivity limit is more relevant than either a limit of detection (LOD) or limit of quantitation (LOQ) calculation - parameters that do not stipulate using a clinically relevant time span for assessing precision (10,560). The FS protocol (10) also stipulates that precision be determined in human sera rather than a commercial QC preparation, because instruments and methods are matrix-sensitive (72,560). Tg IMA methods should have precision determined in TgAb-negative human sera (560) and TgAb-positive human serum pools should be used to determine the precision of Tg methodologies used to measure TgAb-positive specimens - most commonly RIA or LC-MS/MS.
In accord with TSH a generational approach to Tg assay nomenclature has been adopted (1,17). Early Tg RIAs (5) had FS approximating 1 μg/L and were designated "first generation" assays. Currently, some RIAs, IMAs and LC-MS/MS methods still only have first generation functional sensitivity (FS = 0.5-1.0 µg/L) (17,271,274,512,561,562). However, in recent years 2nd generation assays (FS 0.05-0.10 µg/L) have become the standard of care (57,271,274,549,550,561,563). These second-generation tests obviate the need for recombinant human TSH (rhTSH) stimulation, because basal Tg correlates with rhTSH-stimulated Tg (17,57,561). However, the use of a second-generation assay does not eliminate the need for periodic ultrasound examinations, because many histologically confirmed lymph nodes metastases may not secrete enough Tg to be detected (14,563,564).
SERUM Tg REFERENCE RANGES
The adult serum Tg reference range approximates 2-40 µg/L (10,565). Newborn infants have a higher serum Tg that falls to the adult range after two years of age (566). However, most Tg testing is made following surgery (thyroidectomy or lobectomy) for DTC, the Tg reference range is only relevant in the preoperative period (567-570). Different Tg methods may report two-fold differences in numeric values for the same serum specimen (14,274,571). This between-method variability reflects differences in assay standardization as well as the assay specificity for detecting different Tg isoforms in the serum (512,572-576). When evaluating a thyroidectomized patient, the assay reference range should be adjusted for thyroid mass (thyroidectomy versus lobectomy) as well as the TSH status of the patient (10,570).
BETWEEN METHOD Tg BIASES
Thyroglobulin in frozen sera is remarkably stable. The between-run precision for repetitive serum Tg measurements made over 6-12 months (the typical DTC monitoring interval), approximates 10 percent. In contrast, between-method variability can exceed 30 percent (14,274,516,571) despite CRM-457 standardization (584,585). In fact, in some cases different methods can report more than a two-fold difference in Tg for the same serum specimen (14,274,571). This between-method variability significantly exceeds the biologic variability of Tg in normal euthyroid subjects (~16 %) (559,577). This between-method variability reflects matrix differences between methods as well as specificity differences for detecting different Tg isoforms in the serum (45,512,572-574,576).
Some Tg should be detected in all TgAb-negative normal euthyroid subjects when using a second-generation IMA method standardized against the International Reference Preparation CRM-457. Although the intra-individual serum Tg variability is relatively narrow (CV ~15 %) (577), the Tg population reference range is quite broad (2-40 µg/L) (512,565,575,578). It follows that 1 gram of normal thyroid tissue gives rise to ~1.0 µg/L Tg in the circulation, unless TSH is elevated (10,579). Following a lobectomy, euthyroid patients should be evaluated using a mass-adjusted reference range (1.5 - 20 µg/L). The range should be lowered a further 50 percent (0.75 - 10 µg/L) during TSH-suppression (10,570). After thyroidectomy, the typical 1-to-2-gram thyroid remnant (580) would be expected to produce a serum Tg below 2 µg/L (at low-normal TSH) (581,582). By this same reasoning, truly athyreotic patients would be expected to have no Tg detected irrespective of their TSH status (10). However, a rising Tg trend after lobectomy in the absence of recurrent disease is not unexpected due to a compensatory increase in normal remnant tissue (583).
Since TgAb interferes with different methods to differing extents (14,45,586), a false negative TgAb test could also lead to significant between-method differences with the potential to disrupt serial Tg monitoring and negatively impact clinical management (516). Between method variability is the reason current guidelines stress the necessity of using the same Tg method (and preferably the same laboratory) for monitoring Tg trends and the need to re-baseline the Tg level if a change in method becomes necessary (57,587).
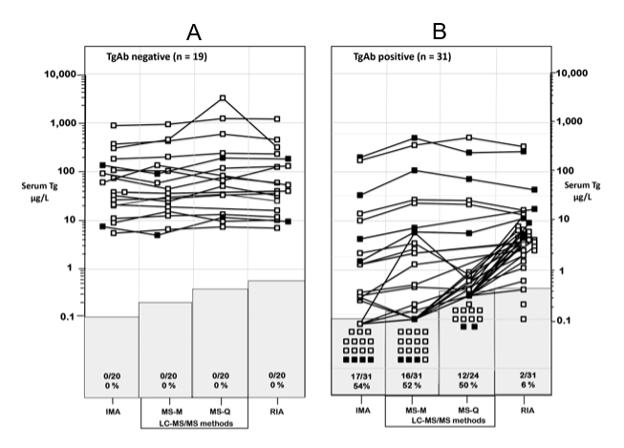
Figure 11. Between-Method Serum Tg Variability in DTC Patients +/- TgAb. Serum Tg measured by different methodologies in patients with distant metastatic DTC who were either TgAb-negative (panel A) or TgAb-positive (panel B). Three Tg methodologies were compared: IMA, LC-MS/MS (MS-M = Mayo; MS-Q = Quest), and RIA. Tg measurements below the assay FS limit are indicated in the shaded areas and expressed as a percentage relative to the total number of tests performed with that method. Patients who died of DTC-related complications are shown by solid symbols. From reference 14.
HIGH-DOSE HOOK EFFECT
Tumor marker tests employing IMA methodology can be prone to so-called "high-dose hook effects", whereby very high antigen concentrations can overwhelm the binding capacity of the monoclonal antibody reagents leading to a falsely normal/low value (9,588-591). Manufacturers have largely overcome hook problems by adopting a two-step procedure, whereby a wash step is used to remove unbound antigen after the first incubation of specimen with the capture monoclonal antibody before introducing the labeled monoclonal during which the signal binds the captured antigen during a second incubation (580). When using IMA methodology, it is the laboratory’s responsibility to determine whether a hook effect is likely to generate falsely normal or low values.
There are two approaches for detecting and overcoming hook effects with Tg IMA methods when an unexpectedly low serum Tg value is encountered for a patient with known metastatic disease: 1) Measure the Tg in the specimen at two dilutions. For example, a hook effect is likely present when the value of the test serum measured at a 1/5 or 1/10 dilution is higher than that obtained with the undiluted specimen. 2) Assess the recovery of added Tg antigen. If a hook effect is present, the Tg result will be inappropriately low.
INTERFERENCES WITH Tg MEASUREMENT
Heterophile Antibody (HAb) Interferences
HAb interferes with Tg IMAs, but not RIA or Tg-LC-MS/MS methodologies (43, 214, 221, 223, 228, 592-595). HAb interferences are thought to reflect the binding of HAb to the monoclonal antibody IMA reagents (murine origin). RIA methods are not prone to HAb interference because their polyclonal antibody reagents (rabbit origin) do not bind human IgG. In most cases HAb interferences are characterized by a false-positive Tg-IMA result (223,228,592), although falsely low Tg IMA results have also been reported (214,596). Recent reports find that Tg LC-MS/MS methodology appears free from HAb interferences (43,595). A presumptive test for HAb interference is a lowering of the analyte value in the presence of a blocking agent (43,201,597). The laboratory cannot proactively test for HAb because specimens are typically sent to the laboratory without clinical information. Physicians should request the laboratory test for HAb interference when an apparently disease-free patient has an unexpectedly high Tg result.
Tg Autoantibody (TgAb) Interference
TgAb interference with Tg measurement remains the major limitation for using Tg as a DTC tumor marker. TgAb has the potential to interfere with Tg measured by each of the current methodologies: IMA, RIA and LC-MS/MS. The prevalence of TgAb in DTC patients approximates 25 percent - twice that of the general population (9,254,522). There appears to be no threshold TgAb concentration that precludes TgAb interference (45,57,386,510,512,521). TgAb is thought to interfere by both in vitro (epitope masking) (45,512,521,598) and/or in vivo mechanisms such as enhanced TgAb-mediated Tg clearance (599-603). High TgAb concentrations do not necessarily interfere, whereas low TgAb may profoundly interfere (9,22,45,521,555,598,604,605). Unfortunately, the recovery approach appears to be unreliable for detecting TgAb interference (512,521,598).
TgAb Interference - In-Vivo Mechanisms. Studies over past decades have suggested that the presence of TgAb enhances Tg metabolic clearance. In 1967 Weigle showed enhanced clearance of endogenously I25-labeled Tg in rabbits, after inducing TgAb by immunizing the animals with an immunogenic Tg preparation (599,603). In humans, Tg and TgAb acute responses to sub-total thyroidectomy have also suggested that TgAb may increase Tg metabolic clearance (603,606). Changes (a rise or fall) in TgAb versus Tg-RIA concentrations have typically been concordant and appropriate for clinical status, whereas the direction of change in Tg-IMA is typically discordant with Tg-RIA and clinical status (45,274,521,556). In general, the change in TgAb concentrations tends to be steeper than for Tg-RIA (521), as would be consistent with TgAb-mediated Tg clearance, perhaps because some TgAbs act as "sweeper" antibodies that facilitate clearance of antigen (602,603,607).
TgAb Interference - In-Vitro Mechanisms. TgAb interferes with Tg measurement in a qualitative, quantitative, and method-dependent manner (22,45,521,608,609). The potential for in vitro interference is multifactorial and depends not only on the assay methodology (IMA, RIA or LC-MS/MS) (39), but also the concentration and epitope specificity of the TgAb secreted by the patient (22,512,610). RIA methodology appears to quantify total Tg (free Tg + TgAb-bound Tg) whereas IMA primarily detects only the free Tg moiety, i.e. Tg molecules with epitopes not masked by TgAb complexing. Steric masking of Tg epitopes is the reason why TgAb interference with IMA methodology is always unidirectional (underestimation) and why a low Tg-IMA/Tg-RIA ratio has been used to indicate TgAb interference (45,521,555,611,612). The recently developed Tg-LC-MS/MS methodology uses trypsin digestion of Tg-TgAb complexes to liberate a proteotypic Tg peptide. This conceptually attractive approach was primarily developed to overcome TgAb interference with IMA methods thereby eliminating falsely low/undetectable Tg-IMA results that can mask disease. However, recent studies report that a high percentage (>40 %) of TgAb-positive DTC patients with structural disease have paradoxically undetectable Tg-LC-MS/MS tests (14,24,43,555,557,558). More studies are needed to determine why LC-MS/MS fails to detect Tg in TgAb-positive DTC patients with disease. Possibilities to investigate include tumor Tg polymorphisms that prevent the production of the Tg-specific tryptic peptide (38), suboptimal trypsinization of Tg-TgAb complexes, or Tg levels that are truly below detection because of increased clearance of Tg-TgAb complexes by the hepatic asialoglycoprotein receptor (599-602).
TgAb interference with Tg-RIA Methodology. Radioimmunoassay (RIA) was the earliest methodology used to measure Tg (5). Thyroglobulin antigen (from serum or added 125I-Tg tracer) competes for a low concentration of polyclonal (PAb) (usually rabbit) Tg antibody. After incubation, the Tg-PAb complex is precipitated by an anti-rabbit second antibody and the serum Tg concentration is quantified from the 125I-Tg in the precipitate. The first Tg-RIAs developed in the 1970s were insensitive (~2 µg/L) (5,613). Over subsequent decades some Tg-RIAs have achieved first generation functional sensitivity (FS = 0.5 µg/L) by using a long (48-hour) pre-incubation before adding a high specific activity 125I-Tg tracer (614,615). The use of a high affinity polyclonal antibody (616) coupled with a species-specific second antibody appears to minimize TgAb interference. Resistance to TgAb interference is evidenced by appropriately normal Tg-RIA values for TgAb-positive euthyroid controls (512) and detectable Tg-RIA in TgAb-positive DTC patients with structural disease (14,555). The clinical performance of this Tg-RIA contrasts with IMA methods that fail to detect Tg in some TgAb-positive normal euthyroid subjects (512), some TgAb-positive Graves' hyperthyroid patients (14,617), or TgAb-positive patients with structural disease (14,512,618). It should be noted that the propensity of TgAb to interfere with Tg-RIA determinations and cause under- or overestimation (546,608) depends on the patient-specific interactions between Tg and TgAb in the specimen and the RIA reagents (609).
TgAb interference with Tg-IMA Methodology. Most Tg testing is currently made by automated IMAs, whereby antigen is captured by two monoclonal antibodies (MAb) that target different epitopes on the Tg protein (619). TgAb interferes with IMA methodology by steric inhibition – i.e. by blocking the epitope(s) necessary for Tg to bind the MAb(s), so that the MAb-Tg-MAb reaction cannot take place and Tg is reported as falsely low or undetectable. This mechanism of epitope masking is supported by timed recovery studies. Clinically, TgAb interference is evident from the paradoxically low/undetectable Tg-IMA seen for TgAb-positive normal controls (512), patients with Graves' hyperthyroidism (14,617), and DTC patients with active disease (Figures 10 and 11) (14,43,555). High Tg concentrations can overwhelm the TgAb binding capacity rendering Tg-IMA concentrations detectable and lessening the degree of interference (45,555). It follows that as Tg concentrations rise, more Tg is free, the influence of TgAb lessens and the discordance between Tg-IMA and Tg-RIA lessens (Figure 11B) (45,555). Although some IMA methods have claimed to overcome TgAb interference by using monoclonal antibodies directed against specific epitopes not involved in thyroid autoimmunity (580), this approach has not overcome TgAb interferences in clinical practice, possibly because less restricted TgAb epitopes are associated with thyroid carcinomas than with autoimmune thyroid conditions (510,517,620).
TgAb Interference with Tg LC-MS/MS. Liquid Chromatography, Tandem Mass Spectrometry (LC-MS/MS) is the newest methodology used to measure Tg. This methodology measures Tg by trypsinizing the Tg-TgAb complexes in the serum to generate a Tg-specific peptide(s) that can be measured by LC-MS/MS (37-39,41,580,621). Most Tg LC-MS/MS methods only have first generation functional sensitivity (FS ~ 0.5 µg/L) (24,39,40) although more sensitive methods are being developed (621). Tg-LC-MS/MS methodology has been shown free from HAb interferences (43,595) and has been promoted as being free from TgAb interference (24,39,40). However, these claims are not supported by clinical studies in which paradoxically undetectable LC-MS/MS Tg tests are seen for many TgAb-positive DTC patients with structural disease (14,24,43,555,557,558). The higher the TgAb, the more likely that no Tg would be detected by LC-MS/MS in patients with disease (558). It currently appears that when TgAb is present LC-MS/MS methodology offers no diagnostic advantage over IMA.
Clinical Utility of TGAb Used as a Surrogate DTC Tumor Marker
The serum TgAb trend has become recognized as a postoperative surrogate DTC tumor-marker. A declining TgAb trend is a good prognostic sign, whereas a stable or rising TgAb may indicate persistent/recurrent disease (23,57,509,519,521,530,531,533,536,612,622-624). The TgAb half-life in blood approximates 10 weeks (522). Following successful surgery (± radioiodine treatment), TgAb typically falls more than 50 percent in first post-operative year and often decreases to <10 percent after 3-4 years eventually becoming undetectable with reduced stimulation of the immune system by lower Tg antigen levels (45,57,274,522,524,530,531,625). The time needed for a TgAb-positive patient to become TgAb-negative in response to successful treatment is inversely related the initial TgAb concentration, perhaps representing the long-lived memory of plasma cells (274,626). Patients exhibiting a TgAb decline of more than 50 percent by the end of the first post-operative year have been shown to have a low recurrence risk (515,531,534,612,627). However, a significant percentage (~5 %) of TgAb-negative patients may develop transient de novo TgAb-positivity in the early post-operative period, presumably in response to Tg antigen released by surgical trauma (532,628,629). A rise in TgAb can also be seen soon after fine needle aspiration (FNA) biopsy (630-632) or more chronically (months) in response to radiolytic damage following radioiodine treatment (22,633,634). However, the 5 percent of DTC patients that display a sustained de novo TgAb appearance are likely to have recurrent disease (Figure 11B) (532,635). These TgAb-negative to TgAb-positive conversions are the reason why guidelines mandate that TgAb be measured with every Tg test (23,57,635). Patients with persistent disease may exhibit only a marginal TgAb decline or have stable, rising or a de novo TgAb appearance (511,521,531-533,612,622). If serum Tg remains detectable after TgAb becomes negative (~3 % of cases), the risk for disease remains (Figure 11A). Since TgAb tests differ in sensitivity and specificity (Figure 10) (23,45,513,514,636) it is essential to measure the serum TgAb trend by the same method, preferably in the same laboratory (23,45,57,482,511,512,514,535,636).
Serum Tg Monitoring of Patients with DTC
Over the past decade, the incidence of DTC has substantially risen with the detection of small thyroid nodules and micropapillary cancers by ultrasound and other anatomic imaging modalities (57,637-640). Although most DTC patients are rendered disease-free by their initial surgery, approximately 15 percent of patients experience recurrences and approximately 5 percent die from disease-related complications (580,641-644). A risk-stratified approach to diagnosis and treatment is now recommended by current guidelines (57).
In most cases, persistent/recurrent disease is detected within the first five post-operative years, although recurrences can occur decades after initial surgery necessitating life-long monitoring for recurrence (642,643). Since most patients have a low pre-test probability for disease, protocols for follow-up need a high negative predictive value (NPV) to eliminate unnecessary testing, as well as a high positive predictive value (PPV) for identifying patients with persistent/recurrent disease. Because Tg testing is generally recognized as being more sensitive for detecting disease than diagnostic 131I whole body scanning (645), biochemical testing (serum Tg. + TgAb) is used in conjunction with periodic ultrasound (57,645). The persistent technical limitations of Tg and TgAb measurements necessitate close physician-laboratory cooperation.
The majority (~75 %) of DTC patients have no Tg antibodies detected (521). In the absence of TgAb, four factors influence the interpretation of serum Tg concentrations: (1) the mass of thyroid tissue present (normal tissue + tumor); (2) The intrinsic ability of the tumor to secrete Tg; (3) the presence of any inflammation of, or injury to, thyroid tissue following fine needle aspiration biopsy, surgery, RAI therapy, or thyroiditis; and (4) the degree of TSH receptor stimulation by TSH, hCG, or TSAb (10). The presence of TgAb necessitates a shift in focus from monitoring serum Tg as the primary tumor-marker, to monitoring the serum TgAb trend as a surrogate tumor-marker (519).
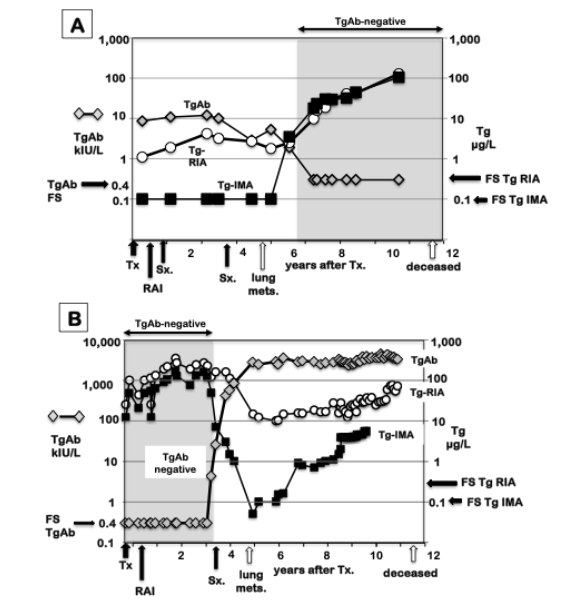
Figure 12. TgAb Effects on Serial Tg IMA and Tg RIA Measurements. Serial TgAb, Tg-RIA and Tg-IMA measurements made in two DTC patients who underwent a change in TgAb status (panel A, positive to negative) or (panel B negative to positive) before death from structural DTC. These cases illustrate why a Tg measurement cannot be interpreted without knowing the TgAb status of the patient (57). The de novo appearance of TgAb (Patient B) either reflects a change in tumor-derived Tg heterogeneity (secretion of a more immunogenic Tg molecule), or recognition of tumor-derived Tg by the immune system. In contrast, TgAb can become undetectable despite the exacerbation of disease (Patient A).
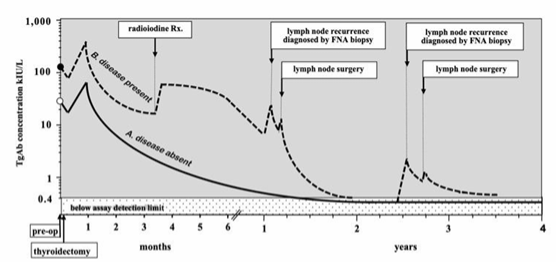
Figure 13. TgAb Trends in Response to Treatment. Typical trends in TgAb following thyroidectomy in patients rendered disease-free by thyroidectomy (pattern A) versus patents with persistent/recurrent disease (pattern B). TgAb levels may rise or become detectable de novo in response to an increase in Tg antigen following surgical injury, lymph node recurrence(s), lymph node resection(s), FNA biopsy of metastatic lymph nodes or radioiodine therapy.
PRE-OPERATIVE Tg MEASUREMENT
An elevated Tg is merely a non-specific indicator of thyroid pathology and cannot be used to diagnose malignancy (568). However, studies have reported that a Tg elevation detected decades before a DTC diagnosis, is a risk factor for thyroid malignancy (567-569,646-648). This suggests that most thyroid cancers secrete Tg protein to an equal or greater degree than normal thyroid tissue, underscoring the importance of using Tg as a DTC tumor marker. Approximately 50 percent of DTC patients have an elevated preoperative serum Tg the highest being seen in follicular > oncocytic (formerly “Hurthle cell cancer”) > papillary thyroid carcinoma (567-569). Up to one-third of tumors may be poor Tg secretors relative to tumor mass, especially BRAF-positive tumors that are associated with reduced expression of Tg protein (649). Although current guidelines do not recommend routine pre-operative serum Tg measurement (57,549,650), some believe that a preoperative serum Tg (drawn before or more than two weeks after FNA) can provide information regarding the tumor’s intrinsic ability to secrete Tg and thus aid with the interpretation of postoperative Tg changes (567-569,648,650). For example, knowing that a tumor is an inefficient Tg secretor could prompt a physician to focus more on anatomic imaging and less on postoperative Tg monitoring (649,651,652).
POST-OPERATIVE Tg MEASUREMENT
Because TSH exerts such a strong influence on serum Tg concentrations it is important to promptly initiate thyroid hormone therapy after surgery to establish a stable post-operative Tg baseline to begin biochemical monitoring. When surgery is followed by RAI treatment it may take time (months) to establish a stable Tg baseline because the Tg rises in response to TSH-stimulation and may be augmented by Tg release from radiolytic damage of the thyroid remnant. Short-term rhTSH stimulation is expected to produce an approximate 10-fold serum Tg elevation (561), whereas chronic endogenous TSH stimulation following thyroid hormone withdrawal results in an approximate 20-fold serum Tg rise (653). Serum Tg measurements performed as early as 6 to 8 weeks after thyroidectomy have been shown to have prognostic value - the higher the serum Tg the greater the risk of persistent/recurrent disease (526, 654, 655). Since the half-life of Tg in the circulation approximates 3 days (656), the acute Tg release resulting from the surgical trauma and healing of surgical margins should largely resolve within the first six months, provided that post-operative thyroid hormone therapy prevents TSH from rising. Patients who receive RAI for remnant ablation may exhibit a slow Tg decline over subsequent years, presumably reflecting the long-term radiolytic destruction of remnant tissue (657,658).
The Tg secretion expected from the ~1 gram of normal remnant tissue left after thyroidectomy (580) is expected to produce a serum Tg concentration ~1.0 µg/L, provided TSH is not elevated (10). A recent study found that in the first six months following thyroidectomy (without RAI treatment) disease-free PTC patients had a serum Tg nadir < 0.5 µg/L when TSH was maintained below 0.5 mIU/L (274,581,582). This is consistent with earlier studies using receiver operator curve (ROC) analysis that found a 6-week serum Tg of <1.0 µg/L, when measured during TSH suppression, had a 98 percent negative predictive value (NPV) for disease (although positive predictive value (PPV) was only 43 percent) (654).
LONG-TERM Tg MONITORING (WITHOUT TSH STIMULATION)
The higher the post-operative serum Tg measured without TSH stimulation, the greater the risk for persistent/recurrent disease (654). If a stable TSH is maintained (≤0.5 mIU/L) (274,582) changes in serum Tg will reflect changes in tumor mass. Under these conditions a rising Tg would be suspicious for tumor recurrence whereas declining Tg levels suggests the absence or regression of disease. When using a sensitive Tg-IMA method, the trend in serum Tg (measured without TSH stimulation) is a more reliable indicator of disease status than using a fixed Tg cutoff value for disease (57,274,548,562,587,654,659-661). It is the degree of Tg elevation, not merely a "detectable" Tg that is the risk factor for disease, since Tg “detectability” varies according to the method used (563,575,578,582). As with other tumor-markers, such as calcitonin, the Tg doubling time (measured without TSH stimulation) is a useful prognostic marker that has an inverse relationship to mortality (252,581,660,662-666). However, between-method variability necessitates that the serum Tg trend be established using the same method, and preferably the same laboratory (Figure 11) (57,587). One approach used to mitigate between-run imprecision and improve the reliability of assessing the Tg trend has been to measure the current specimen concurrently (in the same run) with the patient’s previous archived specimen, thereby eliminating run-to-run variability and increasing the confidence to detect small Tg changes (9,10,587).
SERUM Tg RESPONSES TO TSH STIMULATION
The degree of tumor differentiation determines the presence and density of TSH receptors that in large part determines the magnitude of the serum Tg response to TSH stimulation (667,668). The serum Tg rise in response to endogenous TSH (thyroid hormone withdrawal) is twice that seen with short-term rhTSH stimulation (~20-fold versus ~10-fold, respectively) (386,653,669). Recombinant human TSH (rhTSH) administration was adopted as a standardized approach for stimulating serum Tg into the measurable range of the insensitive first-generation tests (386,549,561,653,669,670). A rhTSH-stimulated serum Tg cut-off of ≥2.0 µg/L, measured 72 hours after the second dose of rhTSH, was found to be a risk factor for disease (653,669). A "positive" rhTSH response had a higher NPV (>95 percent) than the basal Tg measured by an insensitive first-generation test, (553,564,654,671). However, a negative rhTSH test did not guarantee the absence of tumor (653,671). Furthermore, the reliability of adopting a fixed numeric rhTSH-Tg cut-off value for a positive response is problematic, given that different methods can report different numeric Tg values for the same specimen (Figure 11) (14, 512, 575). Other variables include differences in the dose of rhTSH delivered relative to absorption from the injection site as well as the surface area and age of the patient (672,673). One critical variable is the TSH sensitivity of tumor tissues, with poorly differentiated tumors having blunted TSH-mediated Tg responses (649,651,652,668). When using a sensitive second-generation Tg-IMA, an undetectable basal Tg (<0.10 µg/L) had a comparable NPV to rhTSH stimulation and was rarely associated with a "positive" rhTSH-stimulated response (>2.0 µg/L) (561, 563, 575, 674, 675). This would be expected given the strong relationship between basal Tg and rhTSH-stimulated Tg values (553,561,578,676). Once sensitive Tg-IMA methods had become the standard of care, it became apparent that rhTSH-stimulation provided no additional information over and above a basal Tg measured by second generation assay (57, 553, 561, 563, 575, 578, 674-676).
One potential use of rhTSH-stimulated Tg would be to test for HAb interferences. Specifically, when a Tg-IMA value appears clinically inappropriate (usually high), an absent rhTSH-stimulated Tg response would suggest interference that could be confirmed by a blocker tube test (561). An alternative reason for an absent/blunted rhTSH-stimulated response would be the presence of TgAb (578), with TgAb-enhanced clearance of Tg-TgAb complexes (599,602,606).
Tg MEASUREMENT IN FNA NEEDLE WASHOUTS (FNA-Tg)
Because the Tg protein is tissue-specific, the detection of Tg in non-thyroidal tissues or fluids (such as pleural fluid) indicates the presence of metastatic thyroid cancer (677). Struma ovarii is the only (rare) condition in which the Tg in the circulation does not originate from the thyroid (678,679). Cystic thyroid nodules are commonly encountered in clinical practice, the large majority arising from follicular epithelium and the minority from parathyroid epithelium. A high concentration of Tg or parathyroid hormone (PTH) measured in the cyst fluid provides a reliable indicator of the tissue origin of the cyst (thyroid versus parathyroid, respectively), information critical for surgical decision-making (677,680). Lymph node metastases are found in up to 50 percent of patients with papillary cancers but only 20 percent of follicular cancers (681,682). High-resolution ultrasound has now become an important component of postoperative surveillance for recurrence (57,386,669). Although ultrasound characteristics are helpful for distinguishing benign reactive lymph nodes from those suspicious for malignancy, the finding of Tg in the needle washout of a lymph node biopsy has higher diagnostic accuracy than the ultrasound appearance (632,683-691). An FNA needle washout is now widely accepted as a useful adjunctive test that improves the diagnostic sensitivity of a cytological evaluation of a suspicious lymph node or thyroid mass, even in the presence of TgAb (683-687). The current protocol for obtaining FNA-Tg samples recommends rinsing the biopsy needle in 1.0 mL of saline and sending this specimen to the laboratory for Tg analysis. In thyroidectomized patients a common cutoff value for a "positive" FNA-Tg result is 1.0 µg/L, however this cutoff can vary by method and institution (685,686,690-692). For investigations of suspicious lymph nodes in patients with an intact thyroid, a higher FNA-Tg cutoff value (~35-40 µg/L) is recommended (683). There is still controversy whether TgAb interferes with FNA-Tg analyses (528,684). It should be noted that when the serum TgAb concentration is high there can be TgAb contamination of the FNA wash fluid. Although a ~40-fold dilution of TgAb in the wash fluid would be expected, this could still be insufficient to lower TgAb below detection and eliminate the possibility of TgAb interference with the FNA-Tg IMA test producing a falsely low result. The FNA needle wash-out procedure can also be used to detect calcitonin in neck masses of patients with primary and metastatic medullary thyroid cancer (680,693,694). In addition, FNA-PTH determinations may be useful for identifying lymph nodes arising from parathyroid tissue (680).
THYROID SPECIFIC mRNAs USED AS THYROID TUMOR MARKERS
Reverse transcription-polymerase chain reaction (RT-PCR) has been used to detect thyroid-specific mRNAs (Tg, TSHR, TPO and NIS) in the peripheral blood of patients with DTC (579,695-697). Initial studies suggested that circulating Tg mRNA might be employed as a useful tumor marker for thyroid cancer, especially in TgAb-positive patients in whom Tg measurements were subject to TgAb interference (695,698,699). More recently, this approach has been applied to the detection of NIS, TPO, and TSH receptor (TSHR) mRNAs (699,700). Although some studies have suggested that thyroid specific mRNA measurements could be useful for cancer diagnosis and detecting recurrent disease, most studies have concluded that they offer no advantages over sensitive serum Tg measurements (579,699,701). Further, the recent report of false positive Tg mRNA results in patients with congenital athyreosis (702) suggests that Tg mRNA can arise as an assay artifact originating from non-thyroid tissues, or illegitimate transcription (703,704). Conversely, false negative Tg mRNA results have also been observed in patients with documented metastatic disease (705,706). Although Tg, TSHR, NIS and TPO are generally considered “thyroid specific” proteins, mRNAs for these antigens have been detected in non-thyroidal tissues such as lymphocytes, leukocytes, kidney, hepatocytes, brown fat and skin (427,707,708)). Additional sources of variability in mRNA analyses relate to the use of primers that detect splice variants, sample-handling techniques that introduce variability, and difficulties in quantifying the mRNA detected (701,705). The general consensus is that thyroid specific mRNA measurements lack the optimal specificity and practicality to be useful tumor markers (579,699,701). MicroRNA (miRNA) has recently been proposed as an alternate candidate biomarker when Tg measurement is unreliable (709). The growing number of reports of functional TSH receptors and Tg mRNA present in non-thyroidal tissues further suggests that these mRNA measurements will have limited clinical utility in the management of DTC in the future (427,707,708). Further studies in thyroid cancer genomics may yield additional DTC tumor markers with optimal sensitivity and specificity to monitor DTC (710).
REFERENCES
- Spencer CA. Laboratory Thyroid Tests: A Historical Perspective. Thyroid. 2023;33(4):407-419.
- Chaney A. Protein-bound iodine. Advances in clinical chemistry. 1958;Vol. 1(2):81-109.
- Utiger RD. RADIOIMMUNOASSAY OF HUMAN PLASMA THYROTROPIN. J Clin Invest. 1965;44(8):1277-1286.
- Chopra IJ. A radioimmunoassay for measurement of thyroxine in unextracted serum. J Clin Endocrinol Metab.1972;34(6):938-947.
- Van Herle AJ, Uller RP, Matthews NL, Brown J. Radioimmunoassay for measurement of thyroglobulin in human serum. J Clin Invest. 1973;52:1320-1327.
- Chopra IJ. A radioimmunoassay for measurement of 3,3',5'-triiodothyronine (reverse T3). J Clin Invest.1974;54(3):583-592.
- Woodhead J, Addison G, Hales C. The immunoradiometric assay and related techniques. Br Med Bull.1974;30:44-49.
- Spencer CA, LoPresti JS, Patel A, Guttler RB, Eigen A, Shen D, Gray D, Nicoloff JT. Applications of a new chemiluminometric thyrotropin assay to subnormal measurement. J Clin Endocrinol Metab. 1990;70:453-460.
- Spencer CA TM, Kazarosyan M,. Current Status and Performance Goals for Serum Thyroglobulin Assays. Clin Chem. 1996;42(In Press 1/96).
- Baloch Z, Carayon P, Conte-Devolx B, Demers LM, Feldt-Rasmussen U, Henry JF, LiVosli VA, Niccoli-Sire P, John R, Ruf J, Smyth PP, Spencer CA, Stockigt JR. Laboratory medicine practice guidelines. Laboratory support for the diagnosis and monitoring of thyroid disease. Thyroid. 2003;13(1):3-126.
- Dufour DR. Laboratory tests of thyroid function: uses and limitations. Endocrinol Metab Clin North Am.2007;36(3):579-594, v.
- Thienpont LM, Van Uytfanghe K, Beastall G, Faix JD, Ieiri T, Miller WG, Nelson JC, Ronin C, Ross HA, Thijssen JH, Toussaint B. Report of the IFCC Working Group for Standardization of Thyroid Function Tests; part 2: free thyroxine and free triiodothyronine. Clin Chem. 2010;56(6):912-920.
- Van Uytfanghe K, Ehrenkranz J, Halsall D, Hoff K, Loh TP, Spencer CA, Köhrle J. Thyroid Stimulating Hormone and Thyroid Hormones (Triiodothyronine and Thyroxine): An American Thyroid Association-Commissioned Review of Current Clinical and Laboratory Status. Thyroid. 2023;33(9):1013-1028.
- Petrovic I, LoPresti J, Fatemi S, Gianoukakis A, Burman K, Gomez-Lima CJ, Nguyen CT, Spencer CA. Influence of Thyroglobulin Autoantibodies on Thyroglobulin Levels Measured by Different Methodologies: IMA, LC-MS/MS, and RIA. J Clin Endocrinol Metab. 2024;109(12):3254-3263.
- Thienpont LM, Van Uytfanghe K, Poppe K, Velkeniers B. Determination of free thyroid hormones. Best practice & research Clinical endocrinology & metabolism. 2013;27(5):689-700.
- Westbye AB, Aas FE, Kelp O, Dahll LK, Thorsby PM. Analysis of free, unbound thyroid hormones by liquid chromatography-tandem mass spectrometry: A mini-review of the medical rationale and analytical methods. Anal Sci Adv. 2023;4(7-8):244-254.
- Giovanella L, D'Aurizio F, Algeciras-Schimnich A, Görges R, Petranovic Ovcaricek P, Tuttle RM, Visser WE, Verburg FA. Thyroglobulin and thyroglobulin antibody: an updated clinical and laboratory expert consensus. Eur J Endocrinol. 2023;189(2):R11-r27.
- Tozzoli R, D'Aurizio F, Villalta D, Giovanella L. Evaluation of the first fully automated immunoassay method for the measurement of stimulating TSH receptor autoantibodies in Graves' disease. Clin Chem Lab Med. 2017;55(1):58-64.
- Kahaly GJ, Diana T, Olivo PD. TSH Receptor Antibodies: Relevance & Utility. Endocr Pract. 2020;26(1):97-106.
- Lupo MA, Olivo PD, Luffy M, Wolf J, Kahaly GJ. US-based, Prospective, Blinded Study of Thyrotropin Receptor Antibody in Autoimmune Thyroid Disease. J Clin Endocrinol Metab. 2024.
- Jensen EA, Petersen PH, Blaabjerg O, Hansen PS, Brix TH, Hegedüs L. Establishment of reference distributions and decision values for thyroid antibodies against thyroid peroxidase (TPOAb), thyroglobulin (TgAb) and the thyrotropin receptor (TRAb). Clin Chem Lab Med. 2006;44(8):991-998.
- Spencer CA. Clinical review: Clinical utility of thyroglobulin antibody (TgAb) measurements for patients with differentiated thyroid cancers (DTC). J Clin Endocrinol Metab. 2011;96(12):3615-3627.
- Verburg FA, Luster M, Cupini C, Chiovato L, Duntas L, Elisei R, Feldt-Rasmussen U, Rimmele H, Seregni E, Smit JW, Theimer C, Giovanella L. Implications of thyroglobulin antibody positivity in patients with differentiated thyroid cancer: a clinical position statement. Thyroid. 2013;23(10):1211-1225.
- Netzel BC, Grebe SK, Carranza Leon BG, Castro MR, Clark PM, Hoofnagle AN, Spencer CA, Turcu AF, Algeciras-Schimnich A. Thyroglobulin (Tg) Testing Revisited: Tg Assays, TgAb Assays, and Correlation of Results With Clinical Outcomes. J Clin Endocrinol Metab. 2015;100(8):E1074-1083.
- Schussler GC. The thyroxine-binding proteins. Thyroid. 2000;10(2):141-149.
- Pappa T, Ferrara AM, Refetoff S. Inherited defects of thyroxine-binding proteins. Best practice & research Clinical endocrinology & metabolism. 2015;29(5):735-747.
- L Bartalena, D Gallo, E Piantanida. Serum thyroid hormone-binding proteins. In: Encyclopedia of Endocrine Diseases. 2024:442-447.
- Thienpont LM, Van Uytfanghe K, Van Houcke S. Standardization activities in the field of thyroid function tests: a status report. Clin Chem Lab Med. 2010;48(11):1577-1583.
- Thienpont LM, Van Uytfanghe K, Beastall G, Faix JD, Ieiri T, Miller WG, Nelson JC, Ronin C, Ross HA, Thijssen JH, Toussaint B. Report of the IFCC Working Group for Standardization of Thyroid Function Tests; part 1: thyroid-stimulating hormone. Clin Chem. 2010;56(6):902-911.
- Jonklaas J, Sathasivam A, Wang H, Gu J, Burman KD, Soldin SJ. Total and free thyroxine and triiodothyronine: measurement discrepancies, particularly in inpatients. Clin Biochem. 2014;47(13-14):1272-1278.
- Vesper HW, Myers GL, Miller WG. Current practices and challenges in the standardization and harmonization of clinical laboratory tests. Am J Clin Nutr. 2016;104 Suppl 3(Suppl 3):907s-912s.
- Ribera A, Zhang L, Ribeiro C, Vazquez N, Thonkulpitak J, Botelho JC, Danilenko U, van Uytfanghe K, Vesper HW. Practical considerations for accurate determination of free thyroxine by equilibrium dialysis. J Mass Spectrom Adv Clin Lab. 2023;29:9-15.
- Cowper B, Lyle AN, Vesper HW, Van Uytfanghe K, Burns C. Standardisation and harmonisation of thyroid-stimulating hormone measurements: historical, current, and future perspectives. Clin Chem Lab Med.2024;62(5):824-829.
- Thienpont LM, Beastall G, Christofides ND, Faix JD, Ieiri T, Jarrige V, Miller WG, Miller R, Nelson JC, Ronin C, Ross HA, Rottmann M, Thijssen JH, Toussaint B. Proposal of a candidate international conventional reference measurement procedure for free thyroxine in serum. Clin Chem Lab Med. 2007;45(7):934-936.
- Van Houcke SK, Van Uytfanghe K, Shimizu E, Tani W, Umemoto M, Thienpont LM. IFCC international conventional reference procedure for the measurement of free thyroxine in serum: International Federation of Clinical Chemistry and Laboratory Medicine (IFCC) Working Group for Standardization of Thyroid Function Tests (WG-STFT)(1). Clin Chem Lab Med. 2011;49(8):1275-1281.
- Jansen HI, van der Steen R, Brandt A, Olthaar AJ, Vesper HW, Shimizu E, Heijboer AC, Van Uytfanghe K, van Herwaarden AE. Description and validation of an equilibrium dialysis ID-LC-MS/MS candidate reference measurement procedure for free thyroxine in human serum. Clin Chem Lab Med. 2023;61(9):1605-1611.
- Hoofnagle AN, Becker JO, Wener MH, Heinecke JW. Quantification of thyroglobulin, a low-abundance serum protein, by immunoaffinity peptide enrichment and tandem mass spectrometry. Clin Chem. 2008;54(11):1796-1804.
- Hoofnagle AN, Roth MY. Clinical review: improving the measurement of serum thyroglobulin with mass spectrometry. J Clin Endocrinol Metab. 2013;98(4):1343-1352.
- Clarke NJ, Zhang Y, Reitz RE. A novel mass spectrometry-based assay for the accurate measurement of thyroglobulin from patient samples containing antithyroglobulin autoantibodies. Journal of Investigative Medicine: the official publication of the American Federation for Clinical Research. 2012;60(8):1157-1163.
- Kushnir MM, Rockwood AL, Roberts WL, Abraham D, Hoofnagle AN, Meikle AW. Measurement of thyroglobulin by liquid chromatography-tandem mass spectrometry in serum and plasma in the presence of antithyroglobulin autoantibodies. Clin Chem. 2013;59(6):982-990.
- Netzel BC, Grant RP, Hoofnagle AN, Rockwood AL, Shuford CM, Grebe SK. First Steps toward Harmonization of LC-MS/MS Thyroglobulin Assays. Clin Chem. 2016;62(1):297-299.
- Shuford CM, Walters JJ, Holland PM, Sreenivasan U, Askari N, Ray K, Grant RP. Absolute Protein Quantification by Mass Spectrometry: Not as Simple as Advertised. Anal Chem. 2017;89(14):7406-7415.
- Barbesino G, Algeciras-Schimnich A, Bornhorst J. Thyroglobulin Assay Interferences: Clinical Usefulness of Mass-Spectrometry Methods. J Endocr Soc. 2022;7(1):bvac169.
- Thienpont LM, De Brabandere VI, Stöckl D, De Leenheer AP. Development of a new method for the determination of thyroxine in serum based on isotope dilution gas chromatography mass spectrometry. Biol Mass Spectrom.1994;23(8):475-482.
- Spencer C, Petrovic I, Fatemi S. Current thyroglobulin autoantibody (TgAb) assays often fail to detect interfering TgAb that can result in the reporting of falsely low/undetectable serum Tg IMA values for patients with differentiated thyroid cancer. J Clin Endocrinol Metab. 2011;96(5):1283-1291.
- Westbye AB, Aas FE, Dahl SR, Zykova SN, Kelp O, Dahll LK, Thorsby PM. Large method differences for free thyroid hormone assays in the hyperthyroid range can affect assessment of hyperthyroid status: Comparison of Abbott Alinity to Roche Cobas, Siemens Centaur and equilibrium dialysis LC-MS/MS. Clin Biochem. 2023;121-122:110676.
- Stagnaro-Green A, Abalovich M, Alexander E, Azizi F, Mestman J, Negro R, Nixon A, Pearce EN, Soldin OP, Sullivan S, Wiersinga W. Guidelines of the American Thyroid Association for the diagnosis and management of thyroid disease during pregnancy and postpartum. Thyroid. 2011;21(10):1081-1125.
- Alexander EK, Pearce EN, Brent GA, Brown RS, Chen H, Dosiou C, Grobman WA, Laurberg P, Lazarus JH, Mandel SJ, Peeters RP, Sullivan S. 2017 Guidelines of the American Thyroid Association for the Diagnosis and Management of Thyroid Disease During Pregnancy and the Postpartum. Thyroid. 2017;27(3):315-389.
- Garber JR, Cobin RH, Gharib H, Hennessey JV, Klein I, Mechanick JI, Pessah-Pollack R, Singer PA, Woeber KA. Clinical practice guidelines for hypothyroidism in adults: cosponsored by the American Association of Clinical Endocrinologists and the American Thyroid Association. Thyroid. 2012;22(12):1200-1235.
- Pearce SH, Brabant G, Duntas LH, Monzani F, Peeters RP, Razvi S, Wemeau JL. 2013 ETA Guideline: Management of Subclinical Hypothyroidism. Eur Thyroid J. 2013;2(4):215-228.
- Lazarus J, Brown RS, Daumerie C, Hubalewska-Dydejczyk A, Negro R, Vaidya B. 2014 European thyroid association guidelines for the management of subclinical hypothyroidism in pregnancy and in children. Eur Thyroid J. 2014;3(2):76-94.
- Jonklaas J, Bianco AC, Bauer AJ, Burman KD, Cappola AR, Celi FS, Cooper DS, Kim BW, Peeters RP, Rosenthal MS, Sawka AM. Guidelines for the treatment of hypothyroidism: prepared by the american thyroid association task force on thyroid hormone replacement. Thyroid. 2014;24(12):1670-1751.
- Biondi B, Bartalena L, Cooper DS, Hegedüs L, Laurberg P, Kahaly GJ. The 2015 European Thyroid Association Guidelines on Diagnosis and Treatment of Endogenous Subclinical Hyperthyroidism. Eur Thyroid J. 2015;4(3):149-163.
- Ross DS, Burch HB, Cooper DS, Greenlee MC, Laurberg P, Maia AL, Rivkees SA, Samuels M, Sosa JA, Stan MN, Walter MA. 2016 American Thyroid Association Guidelines for Diagnosis and Management of Hyperthyroidism and Other Causes of Thyrotoxicosis. Thyroid. 2016;26(10):1343-1421.
- Gharib H, Papini E, Garber JR, Duick DS, Harrell RM, Hegedüs L, Paschke R, Valcavi R, Vitti P. Medical Guidelines for Clinical Practice for the Diagnosis and Management of Thyroid Nodules--2016 Update. Endocr Pract. 2016;22(5):622-639.
- Francis GL, Waguespack SG, Bauer AJ, Angelos P, Benvenga S, Cerutti JM, Dinauer CA, Hamilton J, Hay ID, Luster M, Parisi MT, Rachmiel M, Thompson GB, Yamashita S. Management Guidelines for Children with Thyroid Nodules and Differentiated Thyroid Cancer. Thyroid. 2015;25(7):716-759.
- Haugen BR, Alexander EK, Bible KC, Doherty GM, Mandel SJ, Nikiforov YE, Pacini F, Randolph GW, Sawka AM, Schlumberger M, Schuff KG, Sherman SI, Sosa JA, Steward DL, Tuttle RM, Wartofsky L. 2015 American Thyroid Association Management Guidelines for Adult Patients with Thyroid Nodules and Differentiated Thyroid Cancer: The American Thyroid Association Guidelines Task Force on Thyroid Nodules and Differentiated Thyroid Cancer. Thyroid. 2016;26(1):1-133.
- Filetti S, Durante C, Hartl D, Leboulleux S, Locati LD, Newbold K, Papotti MG, Berruti A. Thyroid cancer: ESMO Clinical Practice Guidelines for diagnosis, treatment and follow-up†. Ann Oncol. 2019;30(12):1856-1883.
- Bible KC, Kebebew E, Brierley J, Brito JP, Cabanillas ME, Clark TJ, Jr., Di Cristofano A, Foote R, Giordano T, Kasperbauer J, Newbold K, Nikiforov YE, Randolph G, Rosenthal MS, Sawka AM, Shah M, Shaha A, Smallridge R, Wong-Clark CK. 2021 American Thyroid Association Guidelines for Management of Patients with Anaplastic Thyroid Cancer. Thyroid. 2021;31(3):337-386.
- Giannoula E, Exadaktylou P, Melidis C, Koutsouki G, Katsadouros I, Tsangaridi A, Charalambous P, Papadopoulou K, Frangos S, Iakovou I. Real-world applicability of differentiated thyroid cancer guidelines. Hell J Nucl Med.2024;27(2):121-130.
- Bartalena L, Robbins J. Thyroid hormone transport proteins. Clin Lab Med. 1993;13(3):583-598.
- Stockigt JR. Free thyroid hormone measurement. A critical appraisal. Endocrinol Metab Clin North Am.2001;30(2):265-289.
- Murphy BE, Pattee CJ. Determination of Thyroxine Utilizing the Property of Protein-Binding. J Clin Endocrinol Metab. 1964;24:187-196.
- Thienpont LM, Fierens C, De Leenheer AP, Przywara L. Isotope dilution-gas chromatography/mass spectrometry and liquid chromatography/electrospray ionization-tandem mass spectrometry for the determination of triiodo-L-thyronine in serum. Rapid Commun Mass Spectrom. 1999;13(19):1924-1931.
- Soldin SJ, Soukhova N, Janicic N, Jonklaas J, Soldin OP. The measurement of free thyroxine by isotope dilution tandem mass spectrometry. Clin Chim Acta. 2005;358(1-2):113-118.
- Li W, Gao B, Yang T, Tang L, Song D, Li H, Sun K, Xiao P. Development and validation of an isotope dilution-liquid chromatography (ID-LC-MS/MS)-based candidate reference measurement procedure for total 3,3',5-triiodothyronine in human serum. Anal Bioanal Chem. 2025.
- Matsuda M, Sakata S, Komaki T, Nakamura S, Kojima N, Takuno H, Miura K. Effect of 8-anilino-1-naphthalene sulfonic acid (ANS) on the interaction between thyroid hormone and anti-thyroid hormone antibodies. Clin Chim Acta. 1989;185(2):139-146.
- Thienpont LM, Van Uytfanghe K, Marriott J, Stokes P, Siekmann L, Kessler A, Bunk D, Tai S. Feasibility study of the use of frozen human sera in split-sample comparison of immunoassays with candidate reference measurement procedures for total thyroxine and total triiodothyronine measurements. Clin Chem. 2005;51(12):2303-2311.
- Faix JD, Miller WG. Progress in standardizing and harmonizing thyroid function tests. Am J Clin Nutr. 2016;104 Suppl 3(Suppl 3):913s-917s.
- Zhou Q, Li S, Li X, Wang W, Wang Z. Comparability of five analytical systems for the determination of triiodothyronine, thyroxine and thyroid-stimulating hormone. Clin Chem Lab Med. 2006;44(11):1363-1366.
- Algeciras-Schimnich A, Bruns DE, Boyd JC, Bryant SC, La Fortune KA, Grebe SK. Failure of current laboratory protocols to detect lot-to-lot reagent differences: findings and possible solutions. Clin Chem. 2013;59(8):1187-1194.
- Van Houcke SK, Thienpont LM. "Good samples make good assays" – the problem of sourcing clinical samples for a standardization project. Clin Chem Lab Med. 2013;51(5):967-972.
- Lee RH, Spencer CA, Mestman JH, Miller EA, Petrovic I, Braverman LE, Goodwin TM. Free T4 immunoassays are flawed during pregnancy. Am J Obstet Gynecol. 2009;200(3):260.e261-266.
- Wilson KL, Casey BM, McIntire DD, Cunningham FG. Is total thyroxine better than free thyroxine during pregnancy? Am J Obstet Gynecol. 2014;211(2):132.e131-136.
- Korevaar TI, Chaker L, Medici M, de Rijke YB, Jaddoe VW, Steegers EA, Tiemeier H, Visser TJ, Peeters RP. Maternal total T4 during the first half of pregnancy: physiologic aspects and the risk of adverse outcomes in comparison with free T4. Clin Endocrinol (Oxf). 2016;85(5):757-763.
- De Groot L, Abalovich M, Alexander EK, Amino N, Barbour L, Cobin RH, Eastman CJ, Lazarus JH, Luton D, Mandel SJ, Mestman J, Rovet J, Sullivan S. Management of thyroid dysfunction during pregnancy and postpartum: an Endocrine Society clinical practice guideline. J Clin Endocrinol Metab. 2012;97(8):2543-2565.
- Klee GG. Clinical usage recommendations and analytic performance goals for total and free triiodothyronine measurements. Clin Chem. 1996;42(1):155-159.
- Welsh KJ, Soldin SJ. Diagnosis of Endocrine Disease: How reliable are free thyroid and total T3 hormone assays? Eur J Endocrinol. 2016;175(6):R255-r263.
- Ekins R. Measurement of free hormones in blood. Endocr Rev. 1990;11(1):5-46.
- Faix JD. Principles and pitfalls of free hormone measurements. Best practice & research Clinical endocrinology & metabolism. 2013;27(5):631-645.
- Sterling K, Brenner MA. Free thyroxine in human serum: simplified measurement with the aid of magnesium precipitation. J Clin Invest. 1966;45(1):153-163.
- Nelson JC, Weiss RM. The effect of serum dilution on free thyroxine (T4) concentration in the low T4 syndrome of nonthyroidal illness. J Clin Endocrinol Metab. 1985;61(2):239-246.
- Sophianopoulos J, Jerkunica I, Lee CN, Sgoutas D. An improved ultrafiltration method for free thyroxine and triiodothyronine in serum. Clin Chem. 1980;26(1):159-162.
- Wang YS, Hershman JM, Pekary AE. Improved ultrafiltration method for simultaneous measurement of free thyroxin and free triiodothyronine in serum. Clin Chem. 1985;31(4):517-522.
- Weeke J, Boye N, Orskov H. Ultrafiltration method for direct radioimmunoassay measurement of free thyroxine and free tri-iodothyronine in serum. Scand J Clin Lab Invest. 1986;46(4):381-389.
- Romelli PB, Pennisi F, Vancheri L. Measurement of free thyroid hormones in serum by column adsorption chromatography and radioimmunoassay. J Endocrinol Invest. 1979;2(1):25-40.
- Thienpont LM, Van Uytfanghe K, Van Houcke S, Das B, Faix JD, MacKenzie F, Quinn FA, Rottmann M, Van den Bruel A. A Progress Report of the IFCC Committee for Standardization of Thyroid Function Tests. Eur Thyroid J.2014;3(2):109-116.
- Thienpont L, Uytfanghe KV, Grande LD, Reynders D, Das B, Faix J, MacKenzie F, Decallonne B, Hishinuma A, Lapauw B, Taelman P, Crombrugge PV, Bruel AVd, Velkeniers B, Williams P. Harmonization of Serum Thyroid-Stimulating Hormone Measurements Paves the Way for the Adoption of a More Uniform Reference Interval. Clin Chem. 2017;63:1248-1260.
- De Grande LAC, Van Uytfanghe K, Reynders D, Das B, Faix JD, MacKenzie F, Decallonne B, Hishinuma A, Lapauw B, Taelman P, Van Crombrugge P, Van den Bruel A, Velkeniers B, Williams P, Thienpont LM. Standardization of Free Thyroxine Measurements Allows the Adoption of a More Uniform Reference Interval. Clin Chem. 2017;63(10):1642-1652.
- Iitaka M, Kawasaki S, Sakurai S, Hara Y, Kuriyama R, Yamanaka K, Kitahama S, Miura S, Kawakami Y, Katayama S. Serum substances that interfere with thyroid hormone assays in patients with chronic renal failure. Clin Endocrinol (Oxf). 1998;48(6):739-746.
- Moran C, Schoenmakers N, Halsall D, Oddy S, Lyons G, van den Berg S, Gurnell M, Chatterjee K. Approach to the Patient With Raised Thyroid Hormones and Nonsuppressed TSH. J Clin Endocrinol Metab. 2024;109(4):1094-1108.
- Nelson JC, Tomei RT. Direct determination of free thyroxin in undiluted serum by equilibrium dialysis/radioimmunoassay. Clin Chem. 1988;34(9):1737-1744.
- Hopley CJ, Stokes P, Webb KS, Baynham M. The analysis of thyroxine in human serum by an 'exact matching' isotope dilution method with liquid chromatography/tandem mass spectrometry. Rapid Commun Mass Spectrom.2004;18(10):1033-1038.
- Jonklaas J, Kahric-Janicic N, Soldin OP, Soldin SJ. Correlations of free thyroid hormones measured by tandem mass spectrometry and immunoassay with thyroid-stimulating hormone across 4 patient populations. Clin Chem.2009;55(7):1380-1388.
- Van Uytfanghe K, Stöckl D, Ross HA, Thienpont LM. Use of frozen sera for FT4 standardization: investigation by equilibrium dialysis combined with isotope dilution-mass spectrometry and immunoassay. Clin Chem.2006;52(9):1817-1821.
- Yue B, Rockwood AL, Sandrock T, La'ulu SL, Kushnir MM, Meikle AW. Free thyroid hormones in serum by direct equilibrium dialysis and online solid-phase extraction--liquid chromatography/tandem mass spectrometry. Clin Chem. 2008;54(4):642-651.
- Christofides ND, Midgley JE. Inaccuracies in free thyroid hormone measurement by ultrafiltration and tandem mass spectrometry. Clin Chem. 2009;55(12):2228-2229; author reply 2229-2230.
- Tikanoja S. Ultrafiltration devices tested for use in a free thyroxine assay validated by comparison with equilibrium dialysis. Scand J Clin Lab Invest. 1990;50(6):663-669.
- Fritz KS, Wilcox RB, Nelson JC. Quantifying spurious free T4 results attributable to thyroxine-binding proteins in serum dialysates and ultrafiltrates. Clin Chem. 2007;53(5):985-988.
- Tractenberg RE, Jonklaas J, Soldin SJ. Agreement of immunoassay and tandem mass spectrometry in the analysis of cortisol and free t4: interpretation and implications for clinicians. Int J Anal Chem. 2010;2010.
- Tuttlebee J, R B. A comparison of free thyroxine concentration and the free thyroxine index as diagnostic tests of thyroid function. Ann Clin Biochem. 1981;18:88-92.
- Roberts RF, La'ulu SL, Roberts WL. Performance characteristics of seven automated thyroxine and T-uptake methods. Clin Chim Acta. 2007;377(1-2):248-255.
- Nelson JC, Tomei RT. Dependence of the thyroxin/thyroxin-binding globulin (TBG) ratio and the free thyroxin index on TBG concentrations. Clin Chem. 1989;35(4):541-544.
- Stockigt JR, Lim CF. Medications that distort in vitro tests of thyroid function, with particular reference to estimates of serum free thyroxine. Best practice & research Clinical endocrinology & metabolism. 2009;23(6):753-767.
- Cartwright D, O'Shea P, Rajanayagam O, Agostini M, Barker P, Moran C, Macchia E, Pinchera A, John R, Agha A, Ross HA, Chatterjee VK, Halsall DJ. Familial dysalbuminemic hyperthyroxinemia: a persistent diagnostic challenge. Clin Chem. 2009;55(5):1044-1046.
- D'Aurizio F, Kratzsch J, Gruson D, Petranović Ovčariček P, Giovanella L. Free thyroxine measurement in clinical practice: how to optimize indications, analytical procedures, and interpretation criteria while waiting for global standardization. Crit Rev Clin Lab Sci. 2023;60(2):101-140.
- Felicetta JV, Green WL. Value of free thyroxine index. N Engl J Med. 1980;302(26):1480-1481.
- Larsen PR, Alexander NM, Chopra IJ, Hay ID, Hershman JM, Kaplan MM, Mariash CN, Nicoloff JT, Oppenheimer JH, Solomon DH, et al. Revised nomenclature for tests of thyroid hormones and thyroid-related proteins in serum. J Clin Endocrinol Metab. 1987;64(5):1089-1094.
- Litherland PG, Bromage NR, Hall RA. Thyroxine binding globulin (TBG) and thyroxine binding prealbumin (TBPA) measurement, compared with the conventional T3 uptake in the diagnosis of thyroid disease. Clin Chim Acta.1982;122(3):345-352.
- Kundra P, Burman KD. The effect of medications on thyroid function tests. Med Clin North Am. 2012;96(2):283-295.
- Witherspoon LR, Shuler SE, Garcia MM. The triiodothyronine uptake test: an assessment of methods Clin Chem.1981;27:1272-1276.
- Hay ID, Bayer MF, Kaplan MM, Klee GG, Larsen PR, Spencer CA. American Thyroid Association assessment of current free thyroid hormone and thyrotropin measurements and guidelines for future clinical assays. The Committee on Nomenclature of the American Thyroid Association. Clin Chem. 1991;37(11):2002-2008.
- Midgley JE. Direct and indirect free thyroxine assay methods: theory and practice. Clin Chem. 2001;47(8):1353-1363.
- Harpen MD, Lee WN, Siegel JA, Greenfield MA. Serum binding of triiodothyronine: theoretical and practical implications for in vitro triiodothyronine uptake. Endocrinology. 1982;110(5):1732-1739.
- Faix JD, Rosen HN, Velazquez FR. Indirect estimation of thyroid hormone-binding proteins to calculate free thyroxine index: comparison of nonisotopic methods that use labeled thyroxine ("T-uptake"). Clin Chem.1995;41(1):41-47.
- Burr WA, Ramsden DB, Hoffenberg R. Hereditary abnormalities of thyroxine-binding globulin concentration. A study of 19 kindreds with inherited increase or decrease of thyroxine-binding globulin. Q J Med. 1980;49(195):295-313.
- Jensen IW, Faber J. Familial dysalbuminemic hyperthyroxinemia. Acta Med Scand. 1987;221(5):469-473.
- Hoshikawa S, Mori K, Kaise N, Nakagawa Y, Ito S, Yoshida K. Artifactually elevated serum-free thyroxine levels measured by equilibrium dialysis in a pregnant woman with familial dysalbuminemic hyperthyroxinemia. Thyroid.2004;14(2):155-160.
- Ting MJM, Zhang R, Lim EM, Ward BK, Wilson SG, Walsh JP. Familial Dysalbuminemic Hyperthyroxinemia as a Cause for Discordant Thyroid Function Tests. J Endocr Soc. 2021;5(4):bvab012.
- Zouwail SA, O'Toole AM, Clark PM, Begley JP. Influence of thyroid hormone autoantibodies on 7 thyroid hormone assays. Clin Chem. 2008;54(5):927-928.
- Fillée C, Cumps J, Ketelslegers JM. Comparison of three free T4 (FT4) and free T3 (FT3) immunoassays in healthy subjects and patients with thyroid diseases and severe non-thyroidal illnesses. Clin Lab. 2012;58(7-8):725-736.
- Sapin R, Schlienger JL, Gasser F, Noel E, Lioure B, Grunenberger F, Goichot B, Grucker D. Intermethod discordant free thyroxine measurements in bone marrow-transplanted patients. Clin Chem. 2000;46(3):418-422.
- Surks MI, Sievert R. Drugs and thyroid function. N Engl J Med. 1995;333(25):1688-1694.
- Levinson SS, Rieder SV. Parameters affecting a rapid method in which Sephadex is used to determine the percentage of free thyroxine in serum. Clin Chem. 1974;20(12):1568-1572.
- Oppenheimer JH, Squef R, Surks MI, Hauer H. BINDING OF THYROXINE BY SERUM PROTEINS EVALUATED BY EQUILIBRUM DIALYSIS AND ELECTROPHORETIC TECHNIQUES. ALTERATIONS IN NONTHYROIDAL ILLNESS. J Clin Invest. 1963;42(11):1769-1782.
- Snyder SM, Cavalieri RR, Ingbar SH. Simultaneous measurement of percentage free thyroxine and triiodothyronine: comparison of equilibrium dialysis and Sephadex chromatography. J Nucl Med. 1976;17(7):660-664.
- Ross HA, Benraad TJ. Is free thyroxine accurately measurable at room temperature? Clin Chem. 1992;38(6):880-886.
- Uchimura H, Nagataki S, Tabuchi T, MMizuno, Ingbar S. Measurements of free thyroxine: comparison of per cent of free thyroxine in diluted and undiluted sera. J Clin Endocrinol Metab. 1976;42:561-566.
- Witherspoon LR, Shuler SE, Garcia MM, Zollinger LA. Effects of contaminant radioactivity on results of 125I-radioligand assay. Clin Chem. 1979;25(11):1975-1977.
- Midgley JE, Hoermann R. Measurement of total rather than free thyroxine in pregnancy: the diagnostic implications. Thyroid. 2013;23(3):259-261.
- Ma Z, Liu Z, Deng Y, Bai X, Zhou W, Zhang C. Free thyroid hormone: Methods and standardization. Clin Chim Acta. 2025;565:119944.
- Toldy E, Locsei Z, Szabolcs I, Bezzegh A, Kovács GL. Protein interference in thyroid assays: an in vitro study with in vivo consequences. Clin Chim Acta. 2005;352(1-2):93-104.
- van Deventer HE, Mendu DR, Remaley AT, Soldin SJ. Inverse log-linear relationship between thyroid-stimulating hormone and free thyroxine measured by direct analog immunoassay and tandem mass spectrometry. Clin Chem.2011;57(1):122-127.
- Gounden V, Jonklaas J, Soldin SJ. A pilot study: subclinical hypothyroidism and free thyroid hormone measurement by immunoassay and mass spectrometry. Clin Chim Acta. 2014;430:121-124.
- Kratzsch J, Baumann NA, Ceriotti F, Lu ZX, Schott M, van Herwaarden AE, Henriques Vieira JG, Kasapic D, Giovanella L. Global FT4 immunoassay standardization: an expert opinion review. Clin Chem Lab Med.2021;59(6):1013-1023.
- Piketty ML, Bounaud MP, Bounaud JY, Lebtahi R, Valat C, Askienazy S, Begon F, Besnard JC. Multicentre evaluation of a two-step automated enzyme immunoassay of free thyroxine. Eur J Clin Chem Clin Biochem.1992;30(8):485-492.
- Christofides ND, Sheehan CP. Multicenter evaluation of enhanced chemiluminescence labeled-antibody immunoassay (Amerlite-MAB) for free thyroxine. Clin Chem. 1995;41(1):24-31.
- Martel J, Després N, Ahnadi CE, Lachance JF, Monticello JE, Fink G, Ardemagni A, Banfi G, Tovey J, Dykes P, John R, Jeffery J, Grant AM. Comparative multicentre study of a panel of thyroid tests using different automated immunoassay platforms and specimens at high risk of antibody interference. Clin Chem Lab Med. 2000;38(8):785-793.
- Sapin R, d'Herbomez M. Free thyroxine measured by equilibrium dialysis and nine immunoassays in sera with various serum thyroxine-binding capacities. Clin Chem. 2003;49(9):1531-1535.
- Masika LS, Zhao Z, Soldin SJ. Is measurement of TT3 by immunoassay reliable at low concentrations? A comparison of the Roche Cobas 6000 vs. LC-MSMS. Clin Biochem. 2016;49(12):846-849.
- Livingston M, Birch K, Guy M, Kane J, Heald AH. No role for tri-iodothyronine (T3) testing in the assessment of levothyroxine (T4) over-replacement in hypothyroid patients. Br J Biomed Sci. 2015;72(4):160-163.
- Berberoğlu M. Drugs and thyroid interaction. Pediatr Endocrinol Rev. 2003;1 Suppl 2:251-256.
- Brown SJ, Bremner AP, Hadlow NC, Feddema P, Leedman PJ, O'Leary PC, Walsh JP. The log TSH-free T4 relationship in a community-based cohort is nonlinear and is influenced by age, smoking and thyroid peroxidase antibody status. Clin Endocrinol (Oxf). 2016;85(5):789-796.
- Hadlow NC, Rothacker KM, Wardrop R, Brown SJ, Lim EM, Walsh JP. The relationship between TSH and free T₄in a large population is complex and nonlinear and differs by age and sex. J Clin Endocrinol Metab.2013;98(7):2936-2943.
- Chaker L, Korevaar TI, Medici M, Uitterlinden AG, Hofman A, Dehghan A, Franco OH, Peeters RP. Thyroid Function Characteristics and Determinants: The Rotterdam Study. Thyroid. 2016;26(9):1195-1204.
- Jones AM, Honour JW. Unusual results from immunoassays and the role of the clinical endocrinologist. Clin Endocrinol (Oxf). 2006;64(3):234-244.
- Teti C, Nazzari E, Galletti MR, Mandolfino MG, Pupo F, Pesce G, Lillo F, Bagnasco M, Benvenga S. Unexpected Elevated Free Thyroid Hormones in Pregnancy. Thyroid. 2016;26(11):1640-1644.
- Soheilipour F, Fazilaty H, Jesmi F, Gahl WA, Behnam B. First report of inherited thyroxine-binding globulin deficiency in Iran caused by a known de novo mutation in SERPINA7. Mol Genet Metab Rep. 2016;8:13-16.
- Jin HY. Thyroxine binding globulin excess detected by neonatal screening. Ann Pediatr Endocrinol Metab.2016;21(2):105-108.
- Greenberg SM, Ferrara AM, Nicholas ES, Dumitrescu AM, Cody V, Weiss RE, Refetoff S. A novel mutation in the Albumin gene (R218S) causing familial dysalbuminemic hyperthyroxinemia in a family of Bangladeshi extraction. Thyroid. 2014;24(6):945-950.
- Osaki Y, Hayashi Y, Nakagawa Y, Yoshida K, Ozaki H, Fukazawa H. Familial Dysalbuminemic Hyperthyroxinemia in a Japanese Man Caused by a Point Albumin Gene Mutation (R218P). Jpn Clin Med. 2016;7:9-13.
- Cho YY, Song JS, Park HD, Kim YN, Kim HI, Kim TH, Chung JH, Ki CS, Kim SW. First Report of Familial Dysalbuminemic Hyperthyroxinemia With an ALB Variant. Ann Lab Med. 2017;37(1):63-65.
- Pietras SM, Safer JD. Diagnostic confusion attributable to spurious elevation of both total thyroid hormone and thyroid hormone uptake measurements in the setting of autoantibodies: case report and review of related literature. Endocr Pract. 2008;14(6):738-742.
- Massart C, Elbadii S, Gibassier J, Coignard V, Rasandratana A. Anti-thyroxine and anti-triiodothyronine antibody interferences in one-step free triiodothyronine and free thyroxine immunoassays. Clin Chim Acta. 2009;401(1-2):175-176.
- Loh TP, Leong SM, Loke KY, Deepak DS. Spuriously elevated free thyroxine associated with autoantibodies, a result of laboratory methodology: case report and literature review. Endocr Pract. 2014;20(8):e134-139.
- Okosieme OE, Agrawal M, Usman D, Evans C. Method-dependent variation in TSH and FT4 reference intervals in pregnancy: A systematic review. Ann Clin Biochem. 2021;58(5):537-546.
- Osinga JAJ, Nelson SM, Walsh JP, Ashoor G, Palomaki GE, López-Bermejo A, Bassols J, Aminorroaya A, Broeren MAC, Chen L, Lu X, Brown SJ, Veltri F, Huang K, Männistö T, Vafeiadi M, Taylor PN, Tao FB, Chatzi L, Kianpour M, Suvanto E, Grineva EN, Nicolaides KH, D'Alton ME, Poppe KG, Alexander E, Feldt-Rasmussen U, Bliddal S, Popova PV, Chaker L, Visser WE, Peeters RP, Derakhshan A, Vrijkotte TGM, Pop VJM, Korevaar TIM. Defining gestational thyroid dysfunction through modified non-pregnancy reference intervals: an individual participant meta-analysis. J Clin Endocrinol Metab. 2024.
- Osinga JAJ, Derakhshan A, Palomaki GE, Ashoor G, Männistö T, Maraka S, Chen L, Bliddal S, Lu X, Taylor PN, Vrijkotte TGM, Tao FB, Brown SJ, Ghafoor F, Poppe K, Veltri F, Chatzi L, Vaidya B, Broeren MAC, Shields BM, Itoh S, Mosso L, Popova PV, Anopova AD, Kishi R, Aminorroaya A, Kianpour M, López-Bermejo A, Oken E, Pirzada A, Vafeiadi M, Bramer WM, Suvanto E, Yoshinaga J, Huang K, Bassols J, Boucai L, Feldt-Rasmussen U, Grineva EN, Pearce EN, Alexander EK, Pop VJM, Nelson SM, Walsh JP, Peeters RP, Chaker L, Nicolaides KH, D'Alton ME, Korevaar TIM. TSH and FT4 Reference Intervals in Pregnancy: A Systematic Review and Individual Participant Data Meta-Analysis. J Clin Endocrinol Metab. 2022;107(10):2925-2933.
- Chan S, Boelaert K. Optimal management of hypothyroidism, hypothyroxinaemia and euthyroid TPO antibody positivity preconception and in pregnancy. Clin Endocrinol (Oxf). 2015;82(3):313-326.
- Varner MW, Mele L, Casey BM, Peaceman AM, Reddy UM, Wapner RJ, Thorp JM, Saade GR, Tita ATN, Rouse DJ, Sibai BM, Costantine MM, Mercer BM, Caritis SN. Progression of Gestational Subclinical Hypothyroidism and Hypothyroxinemia to Overt Hypothyroidism After Pregnancy: Pooled Analysis of Data from Two Randomized Controlled Trials. Thyroid. 2024;34(9):1171-1176.
- Oguz A, Tuzun D, Sahin M, Usluogullari AC, Usluogullari B, Celik A, Gul K. Frequency of isolated maternal hypothyroxinemia in women with gestational diabetes mellitus in a moderately iodine-deficient area. Gynecol Endocrinol. 2015;31(10):792-795.
- Haddow JE, Craig WY, Neveux LM, Palomaki GE, Lambert-Messerlian G, Malone FD, D'Alton ME. Free Thyroxine During Early Pregnancy and Risk for Gestational Diabetes. PloS one. 2016;11(2):e0149065.
- Yang S, Shi FT, Leung PC, Huang HF, Fan J. Low Thyroid Hormone in Early Pregnancy Is Associated With an Increased Risk of Gestational Diabetes Mellitus. J Clin Endocrinol Metab. 2016;101(11):4237-4243.
- Berta E, Samson L, Lenkey A, Erdei A, Cseke B, Jenei K, Major T, Jakab A, Jenei Z, Paragh G, Nagy EV, Bodor M. Evaluation of the thyroid function of healthy pregnant women by five different hormone assays. Pharmazie.2010;65(6):436-439.
- Laurberg P, Andersen SL, Hindersson P, Nohr EA, Olsen J. Dynamics and Predictors of Serum TSH and fT4 Reference Limits in Early Pregnancy: A Study Within the Danish National Birth Cohort. J Clin Endocrinol Metab.2016;101(6):2484-2492.
- Price A, Obel O, Cresswell J, Catch I, Rutter S, Barik S, Heller SR, Weetman AP. Comparison of thyroid function in pregnant and non-pregnant Asian and western Caucasian women. Clin Chim Acta. 2001;308(1-2):91-98.
- Dhatt GS, Jayasundaram R, Wareth LA, Nagelkerke N, Jayasundaram K, Darwish EA, Lewis A. Thyrotrophin and free thyroxine trimester-specific reference intervals in a mixed ethnic pregnant population in the United Arab Emirates. Clin Chim Acta. 2006;370(1-2):147-151.
- La'ulu SL, Roberts WL. Ethnic differences in first-trimester thyroid reference intervals. Clin Chem. 2011;57(6):913-915.
- Korevaar TI, Medici M, de Rijke YB, Visser W, de Muinck Keizer-Schrama SM, Jaddoe VW, Hofman A, Ross HA, Visser WE, Hooijkaas H, Steegers EA, Tiemeier H, Bongers-Schokking JJ, Visser TJ, Peeters RP. Ethnic differences in maternal thyroid parameters during pregnancy: the Generation R study. J Clin Endocrinol Metab.2013;98(9):3678-3686.
- Medici M, Korevaar TI, Visser WE, Visser TJ, Peeters RP. Thyroid function in pregnancy: what is normal? Clin Chem. 2015;61(5):704-713.
- Antonangeli L, Maccherini D, Cavaliere R, Di Giulio C, Reinhardt B, Pinchera A, Aghini-Lombardi F. Comparison of two different doses of iodide in the prevention of gestational goiter in marginal iodine deficiency: a longitudinal study. Eur J Endocrinol. 2002;147(1):29-34.
- Moleti M, Di Bella B, Giorgianni G, Mancuso A, De Vivo A, Alibrandi A, Trimarchi F, Vermiglio F. Maternal thyroid function in different conditions of iodine nutrition in pregnant women exposed to mild-moderate iodine deficiency: an observational study. Clin Endocrinol (Oxf). 2011;74(6):762-768.
- Shi X, Han C, Li C, Mao J, Wang W, Xie X, Li C, Xu B, Meng T, Du J, Zhang S, Gao Z, Zhang X, Fan C, Shan Z, Teng W. Optimal and safe upper limits of iodine intake for early pregnancy in iodine-sufficient regions: a cross-sectional study of 7190 pregnant women in China. J Clin Endocrinol Metab. 2015;100(4):1630-1638.
- Männistö T, Hartikainen AL, Vääräsmäki M, Bloigu A, Surcel HM, Pouta A, Järvelin MR, Ruokonen A, Suvanto E. Smoking and early pregnancy thyroid hormone and anti-thyroid antibody levels in euthyroid mothers of the Northern Finland Birth Cohort 1986. Thyroid. 2012;22(9):944-950.
- Lem AJ, de Rijke YB, van Toor H, de Ridder MA, Visser TJ, Hokken-Koelega AC. Serum thyroid hormone levels in healthy children from birth to adulthood and in short children born small for gestational age. J Clin Endocrinol Metab. 2012;97(9):3170-3178.
- Chaler EA, Fiorenzano R, Chilelli C, Llinares V, Areny G, Herzovich V, Maceiras M, Lazzati JM, Mendioroz M, Rivarola MA, Belgorosky A. Age-specific thyroid hormone and thyrotropin reference intervals for a pediatric and adolescent population. Clin Chem Lab Med. 2012;50(5):885-890.
- La'ulu SL, Rasmussen KJ, Straseski JA. Pediatric Reference Intervals for Free Thyroxine and Free Triiodothyronine by Equilibrium Dialysis-Liquid Chromatography-Tandem Mass Spectrometry. J Clin Res Pediatr Endocrinol. 2016;8(1):26-31.
- Soldin SJ, Cheng LL, Lam LY, Werner A, Le AD, Soldin OP. Comparison of FT4 with log TSH on the Abbott Architect ci8200: Pediatric reference intervals for free thyroxine and thyroid-stimulating hormone. Clin Chim Acta.2010;411(3-4):250-252.
- Loh TP, Sethi SK, Metz MP. Paediatric reference interval and biological variation trends of thyrotropin (TSH) and free thyroxine (T4) in an Asian population. J Clin Pathol. 2015;68(8):642-647.
- Lauffer P, Heinen CA, Goorsenberg AWM, Malekzadeh A, Henneman P, Heijboer AC, Zwaveling-Soonawala N, Boelen A, van Trotsenburg ASP. Analysis of Serum Free Thyroxine Concentrations in Healthy Term Neonates Underlines Need for Local and Laboratory-Specific Reference Interval: A Systematic Review and Meta-Analysis of Individual Participant Data. Thyroid. 2024;34(5):559-565.
- Haugen BR. Drugs that suppress TSH or cause central hypothyroidism. Best practice & research Clinical endocrinology & metabolism. 2009;23(6):793-800.
- Koulouri O, Moran C, Halsall D, Chatterjee K, Gurnell M. Pitfalls in the measurement and interpretation of thyroid function tests. Best practice & research Clinical endocrinology & metabolism. 2013;27(6):745-762.
- Fliers E, Boelen A. An update on non-thyroidal illness syndrome. J Endocrinol Invest. 2021;44(8):1597-1607.
- Hamblin PS, SA. D, Mohr VS, Le Grand B, Lim CF, Tuxen DV, Topliss DJ, Stockigt JR. Relationship between thyrotropin and thyroxine changes during recovery from severe hypothyroxinemia of critical illness. J Clin Endocrinol Metab. 1986;62:717-722.
- Spencer CA, Eigen A, Shen D, Duda M, Qualls S, Weiss S, Nicoloff JT. Specificity of sensitive assays of thyrotropin (TSH) used to screen for thyroid disease in hospitalized patients. Clin Chem. 1987;33:1391-1396.
- de Vries EM, Fliers E, Boelen A. The molecular basis of the non-thyroidal illness syndrome. J Endocrinol.2015;225(3):R67-81.
- Moura Neto A, Zantut-Wittmann DE. Abnormalities of Thyroid Hormone Metabolism during Systemic Illness: The Low T3 Syndrome in Different Clinical Settings. Int J Endocrinol. 2016;2016:2157583.
- LoPresti J, Patil K. Assessing thyroid function in hospitalized patients. New York: Springer.
- Rotmensch S, Cole LA. False diagnosis and needless therapy of presumed malignant disease in women with false-positive human chorionic gonadotropin concentrations. Lancet. 2000;355(9205):712-715.
- Ballieux BE, Weijl NI, Gelderblom H, van Pelt J, Osanto S. False-positive serum human chorionic gonadotropin (HCG) in a male patient with a malignant germ cell tumor of the testis: a case report and review of the literature. Oncologist. 2008;13(11):1149-1154.
- Dimeski G. Interference testing. Clin Biochem Rev. 2008;29 Suppl 1(Suppl 1):S43-48.
- Henry N, Sebe P, Cussenot O. Inappropriate treatment of prostate cancer caused by heterophilic antibody interference. Nat Clin Pract Urol. 2009;6(3):164-167.
- Georges A, Charrié A, Raynaud S, Lombard C, Corcuff JB. Thyroxin overdose due to rheumatoid factor interferences in thyroid-stimulating hormone assays. Clin Chem Lab Med. 2011;49(5):873-875.
- Pishdad GR, Pishdad P, Pishdad R. The effect of glucocorticoid therapy on a falsely raised thyrotropin due to heterophilic antibodies. Thyroid. 2013;23(12):1657-1658.
- Favresse J, Burlacu MC, Maiter D, Gruson D. Interferences With Thyroid Function Immunoassays: Clinical Implications and Detection Algorithm. Endocr Rev. 2018;39(5):830-850.
- Ghazal K, Brabant S, Prie D, Piketty ML. Hormone Immunoassay Interference: A 2021 Update. Ann Lab Med.2022;42(1):3-23.
- Campi I, Dell'Acqua M, Stellaria Grassi E, Cristina Vigone M, Persani L. Unusual causes of hyperthyrotropinemia and differential diagnosis of primary hypothyroidism: a revised diagnostic flowchart. Eur Thyroid J. 2023;12(4).
- Ismail Y, Ismail AA, Ismail AA. Erroneous laboratory results: what clinicians need to know. Clin Med (Lond).2007;7(4):357-361.
- Sturgeon CM, Viljoen A. Analytical error and interference in immunoassay: minimizing risk. Ann Clin Biochem.2011;48(Pt 5):418-432.
- Gulbahar O, Konca Degertekin C, Akturk M, Yalcin MM, Kalan I, Atikeler GF, Altinova AE, Yetkin I, Arslan M, Toruner F. A Case With Immunoassay Interferences in the Measurement of Multiple Hormones. J Clin Endocrinol Metab. 2015;100(6):2147-2153.
- Massart C, Corcuff JB, Bordenave L. False-positive results corrected by the use of heterophilic antibody-blocking reagent in thyroglobulin immunoassays. Clin Chim Acta. 2008;388(1-2):211-213.
- King RI, Florkowski CM. How paraproteins can affect laboratory assays: spurious results and biological effects. Pathology. 2010;42(5):397-401.
- LeGatt DF, Higgins TN. Paraprotein interference in immunoassays. Ther Drug Monit. 2015;37(3):417.
- Mandal K, Ashorobi D, Lee A, Liao H, Kumar SC, Rosenthal DS. Factitiously Elevated Total Triiodothyronine in a Euthyroid Patient with Multiple Myeloma. Case Rep Endocrinol. 2021;2021:8479193.
- Sarkar R. A simple method to overcome paraproteinemic interferences in chemistry and immunoassays. Lab Med.2024.
- Sapin R, D'Herbomez M, Schlienger JL. Free thyroxine measured with equilibrium dialysis and nine immunoassays decreases in late pregnancy. Clin Lab. 2004;50(9-10):581-584.
- Anckaert E, Poppe K, Van Uytfanghe K, Schiettecatte J, Foulon W, Thienpont LM. FT4 immunoassays may display a pattern during pregnancy similar to the equilibrium dialysis ID-LC/tandem MS candidate reference measurement procedure in spite of susceptibility towards binding protein alterations. Clin Chim Acta. 2010;411(17-18):1348-1353.
- Guven S, Alver A, Mentese A, Ilhan FC, Calapoglu M, Unsal MA. The novel ischemia marker 'ischemia-modified albumin' is increased in normal pregnancies. Acta Obstet Gynecol Scand. 2009;88(4):479-482.
- Cameron SJ, Hagedorn JC, Sokoll LJ, Caturegli P, Ladenson PW. Dysprealbuminemic hyperthyroxinemia in a patient with hyperthyroid graves disease. Clin Chem. 2005;51(6):1065-1069.
- Sapin R, Gasser F, Schlienger JL. Familial dysalbuminemic hyperthyroxinemia and thyroid hormone autoantibodies: interference in current free thyroid hormone assays. Horm Res. 1996;45(3-5):139-141.
- Kragh-Hansen U, Galliano M, Minchiotti L. Clinical, Genetic, and Protein Structural Aspects of Familial Dysalbuminemic Hyperthyroxinemia and Hypertriiodothyroninemia. Front Endocrinol (Lausanne). 2017;8:297.
- Refetoff S, Scherberg NH, Yuan C, Wu W, Wu Z, McPhaul MJ. Free Thyroxine Concentrations in Sera of Individuals with Familial Dysalbuminemic Hyperthyroxinemia: A Comparison of Three Methods of Measurement. Thyroid. 2020;30(1):37-41.
- DeCosimo DR, Fang SL, Braverman LE. Prevalence of familial dysalbuminemic hyperthyroxinemia in Hispanics. Ann Intern Med. 1987;107(5):780-781.
- Giovanella L, Keller F, Ceriani L, Tozzoli R. Heterophile antibodies may falsely increase or decrease thyroglobulin measurement in patients with differentiated thyroid carcinoma. Clin Chem Lab Med. 2009;47(8):952-954.
- Bolstad N, Warren DJ, Nustad K. Heterophilic antibody interference in immunometric assays. Best practice & research Clinical endocrinology & metabolism. 2013;27(5):647-661.
- Weber TH, Käpyaho KI, Tanner P. Endogenous interference in immunoassays in clinical chemistry. A review. Scand J Clin Lab Invest Suppl. 1990;201:77-82.
- Levinson SS, Miller JJ. Towards a better understanding of heterophile (and the like) antibody interference with modern immunoassays. Clin Chim Acta. 2002;325(1-2):1-15.
- Bjerner J, Olsen KH, Børmer OP, Nustad K. Human heterophilic antibodies display specificity for murine IgG subclasses. Clin Biochem. 2005;38(5):465-472.
- Ellis MJ, Livesey JH. Techniques for identifying heterophile antibody interference are assay specific: study of seven analytes on two automated immunoassay analyzers. Clin Chem. 2005;51(3):639-641.
- Sun HG, Xu XP, He LQ. Pseudo Elevation of TSH and ACTH Caused by Heterophilic Antibodies: a Case Report and Literature Review. Clin Lab. 2024;70(6).
- Astarita G, Gutiérrez S, Kogovsek N, Mormandi E, Otero P, Calabrese C, Alcaraz G, Vázquez A, Abalovich M. False positive in the measurement of thyroglobulin induced by rheumatoid factor. Clin Chim Acta. 2015;447:43-46.
- Mongolu S, Armston AE, Mozley E, Nasruddin A. Heterophilic antibody interference affecting multiple hormone assays: Is it due to rheumatoid factor? Scand J Clin Lab Invest. 2016;76(3):240-242.
- Preissner CM, O'Kane DJ, Singh RJ, Morris JC, Grebe SKG. Phantoms in the assay tube; Heterophile antibody interferences in serum thyroglobulin assays. J Clin Endocrinol Metab. 2003;88:3069-3074.
- Ghosh S, Howlett M, Boag D, Malik I, Collier A. Interference in free thyroxine immunoassay. Eur J Intern Med.2008;19(3):221-222.
- Preissner CM, Dodge LA, O'Kane DJ, Singh RJ, Grebe SK. Prevalence of heterophilic antibody interference in eight automated tumor marker immunoassays. Clin Chem. 2005;51(1):208-210.
- Marks V. False-positive immunoassay results: a multicenter survey of erroneous immunoassay results from assays of 74 analytes in 10 donors from 66 laboratories in seven countries. Clin Chem. 2002;48(11):2008-2016.
- Koshida S, Asanuma K, Kuribayashi K, Goto M, Tsuji N, Kobayashi D, Tanaka M, Watanabe N. Prevalence of human anti-mouse antibodies (HAMAs) in routine examinations. Clin Chim Acta. 2010;411(5-6):391-394.
- Verburg FA, Wäschle K, Reiners C, Giovanella L, Lentjes EG. Heterophile antibodies rarely influence the measurement of thyroglobulin and thyroglobulin antibodies in differentiated thyroid cancer patients. Horm Metab Res. 2010;42(10):736-739.
- Nakano K, Yasuda K, Shibuya H, Moriyama T, Kahata K, Shimizu C. Transient human anti-mouse antibody generated with immune enhancement in a carbohydrate antigen 19-9 immunoassay after surgical resection of recurrent cancer. Ann Clin Biochem. 2016;53(Pt 4):511-515.
- Gessl A, Blueml S, Bieglmayer C, Marculescu R. Anti-ruthenium antibodies mimic macro-TSH in electrochemiluminescent immunoassay. Clin Chem Lab Med. 2014;52(11):1589-1594.
- Rulander NJ, Cardamone D, Senior M, Snyder PJ, Master SR. Interference from anti-streptavidin antibody. Arch Pathol Lab Med. 2013;137(8):1141-1146.
- Dahll LK, Haave EM, Dahl SR, Aas FE, Thorsby PM. Endogenous anti-streptavidin antibodies causing erroneous laboratory results more common than anticipated. Scand J Clin Lab Invest. 2021;81(2):92-103.
- Vos MJ, Rondeel JMM, Mijnhout GS, Endert E. Immunoassay interference caused by heterophilic antibodies interacting with biotin. Clin Chem Lab Med. 2017;55(6):e122-e126.
- Ylli D, Soldin SJ, Stolze B, Wei B, Nigussie G, Nguyen H, Mendu DR, Mete M, Wu D, Gomes-Lima CJ, Klubo-Gwiezdzinska J, Burman KD, Wartofsky L. Biotin Interference in Assays for Thyroid Hormones, Thyrotropin and Thyroglobulin. Thyroid. 2021;31(8):1160-1170.
- McBride M, Dasgupta A. Technical Note: Approach to Identify and Eliminate Biotin Interference in Thyroid Function Tests Using Beckman DXI 800 Analyzer by Taking Advantage of Assay Harmonization with Alinity i Analyzer. Ann Clin Lab Sci. 2023;53(3):482-484.
- McBride M, Dasgupta A. Significant Interference of Biotin in Thyroid Function Tests Using Beckman Analyzer: How to Identify such Interferences? Ann Clin Lab Sci. 2023;53(1):130-133.
- Countryman B, McBride M, McCracken T, Dasgupta A. Effect of biotin on currently used Beckman thyroglobulin assay and newly reformulated thyroglobulin assay not affected by biotin. Am J Clin Pathol. 2024.
- Tang M, Meng X, Ni J, Liu X, Wang X, Li Y, Chai Y, Kou C, Zhang L, Zhang H. The interference of anti-TSH autoantibody on clinical TSH detection. Front Endocrinol (Lausanne). 2024;15:1289923.
- Hattori N, Ishihara T, Matsuoka N, Saito T, Shimatsu A. Anti-Thyrotropin Autoantibodies in Patients with Macro-Thyrotropin and Long-Term Changes in Macro-Thyrotropin and Serum Thyrotropin Levels. Thyroid.2017;27(2):138-146.
- Chiardi I, Rotondi M, Cantù M, Keller F, Trimboli P. Macro-TSH: An Uncommon Explanation for Persistent TSH Elevation That Thyroidologists Have to Keep in Mind. J Pers Med. 2023;13(10).
- Al-Bahadili H, Powers Carson J, Markov A, Jasim S. The Complex Web of Interferences with Thyroid Function Tests. Endocr Pract. 2025;31(1):92-101.
- Gangemi S, Saitta S, Lombardo G, Patafi M, Benvenga S. Serum thyroid autoantibodies in patients with idiopathic either acute or chronic urticaria. J Endocrinol Invest. 2009;32(2):107-110.
- Colucci R, Lotti F, Dragoni F, Arunachalam M, Lotti T, Benvenga S, Moretti S. High prevalence of circulating autoantibodies against thyroid hormones in vitiligo and correlation with clinical and historical parameters of patients. Br J Dermatol. 2014;171(4):786-798.
- Hattori N, Aisaka K, Yamada A, Matsuda T, Shimatsu A. Prevalence and Pathogenesis of Macro-Thyrotropin in Neonates: Analysis of Umbilical Cord Blood from 939 Neonates and Their Mothers. Thyroid. 2023;33(1):45-52.
- Newman JD, Bergman PB, Doery JC, Balazs ND. Factitious increase in thyrotropin in a neonate caused by a maternally transmitted interfering substance. Clin Chem. 2006;52(3):541-542.
- Benvenga S, Ordookhani A, Pearce EN, Tonacchera M, Azizi F, Braverman LE. Detection of circulating autoantibodies against thyroid hormones in an infant with permanent congenital hypothyroidism and her twin with transient congenital hypothyroidism: possible contribution of thyroid hormone autoantibodies to neonatal and infant hypothyroidism. J Pediatr Endocrinol Metab. 2008;21(10):1011-1020.
- Rix M, Laurberg P, Porzig C, Kristensen SR. Elevated thyroid-stimulating hormone level in a euthyroid neonate caused by macro thyrotropin-IgG complex. Acta Paediatr. 2011;100(9):e135-137.
- Ni J, Long Y, Zhang L, Yang Q, Kou C, Li S, Li J, Zhang H. High prevalence of thyroid hormone autoantibody and low rate of thyroid hormone detection interference. J Clin Lab Anal. 2022;36(1):e24124.
- Giovanella L, Dorizzi RM, Keller F. A hypothyroid patient with increased free thyroid hormones. Clin Chem Lab Med. 2008;46(11):1650-1651.
- van der Watt G, Haarburger D, Berman P. Euthyroid patient with elevated serum free thyroxine. Clin Chem.2008;54(7):1239-1241.
- Nicoloff J SC. Use and misuse of the sensitive thyrotropin assays. J Clin Endocrinol Metab. 1990;71:553-558.
- Zhang X, Higuchi T, Tomonaga H, Lamid-Ochir O, Bhattarai A, Nguyen-Thu H, Taketomi-Takahashi A, Hirasawa H, Tsushima Y. Early detection of progressive disease using thyroglobulin doubling-time in metastatic differentiated thyroid carcinoma treated with radioactive iodine. Nucl Med Commun. 2020;41(4):350-355.
- Biondi B. TSH Suppression in Differentiated Thyroid Cancer Patients. Still More Questions than Answers after 30 Years. Thyroid. 2024;34(6):671-673.
- Hollowell JG, Staehling NW, Hannon WH, Flanders WD, Gunter EW, Spencer CA, Braverman LE. Serum thyrotropin, thyroxine, and thyroid antibodies in the United States population (1988 to 1994): NHANES III. J Clin Endocrinol Metab. 2002;87:489-499.
- De Grande LA, Van Uytfanghe K, Thienpont LM. A Fresh Look at the Relationship between TSH and Free Thyroxine in Cross-Sectional Data. Eur Thyroid J. 2015;4(1):69-70.
- Spencer CA, Nicoloff JT. Improved radioimmunoassay for human TSH. Clin Chim Acta. 1980;108:415-424.
- Odell WD, Wilber JF, Paul WE. Radioimmunoassay of thyrotropin in human serum. J Clin Endocrinol Metab.1965;25(9):1179-1188.
- Hershman J, Pittman J. Utility of the radioimmunoassay of serum thyrotrophin in man Ann Intern Med.1971;74:481-490.
- Haigler E, Pittman J, Hershman J, Baugh C. Direct evaluation of pituitary Thyrotropin reserve utilizing synthetic Thyrotropin Releasing Hormone. J Clin Endocrinol Metab. 1971;33:573-581.
- Hall R, Ormston B, Besser G, Cryer R. The Thyrotropin-Releasing Hormone test in diseases of the pituitary and hypothalamus. Lancet. 1972;1:7754-7765.
- Spencer CA, Schwarzbein D, Guttler RB, LoPresti JS, Nicoloff JT. TRH stimulation test responses employing third and fourth generation TSH assays. J Clin Endocrinol Metab. 1993;76:494-498.
- Miles L, CN H. Labeled antibodies and immunological assay systems. Nature. 1968;219:186-189.
- Köhler G, Milstein C. Continuous cultures of fused cells secreting antibody of predefined specificity. Nature.1975;256(5517):495-497.
- García de la Rosa I, Feal Carballo S, Almenares Guasch PR, Segura González MT, Pupo Infante M, Frómeta Suárez A, Martínez Beltrán L, Quintana Guerra JM, Lafita Delfino Y, Morejón García G, Pérez Morás PL, Hernández Pérez L, Castells Martínez EM. Generation and characterization of monoclonal antibodies against thyroid-stimulating hormone for newborn screening of congenital hypothyroidism. J Immunoassay Immunochem.2019;40(4):350-366.
- Seth J, Kellett H, Caldwell G, Sweeting V, Beckett G, Gow S, Toft A. A sensitive immunoradiometric assay for serum thyroid stimulating hormone: a replacement for the thyrotropin releasing test. Br Med J. 1984;289:1334-1336.
- Spencer C, Schwarzbein D, Guttler R, LoPresti J, Nicoloff J. TRH stimulation test responses employing 3rd. and 4th. generation TSH assay technology. J Clin Endocrinol Metab. 1993;76:494-499.
- Spencer C, Takeuchi M, Kazarosyan M. Current status and performance goals for serum thyrotropin (TSH) assays. Clinical Chemistry. 1996;42(1):141-145.
- Taimela E, R T, P K, Nuutila P FJ, S T, SL K, M V, K I. Ability of two new thyrotropin (TSH) assays to separate hyperthyroid patients from euthyroid patients with low TSH. Clin Chem. 1994;40:101-105.
- Moussallieh FM, Ranaivosoa MK, Romain S, Reix N. Analytical validation of two second generation thyroglobulin immunoassays (Roche and Thermo Fisher). Clin Chem Lab Med. 2018;56(12):e302-e305.
- Owen WE, Gantzer ML, Lyons JM, Rockwood AL, Roberts WL. Functional sensitivity of seven automated thyroid stimulating hormone immunoassays. Clin Chim Acta. 2011;412(23-24):2336-2339.
- Giovanella L, Feldt-Rasmussen U, Verburg FA, Grebe SK, Plebani M, Clark PM. Thyroglobulin measurement by highly sensitive assays: focus on laboratory challenges. Clin Chem Lab Med. 2015;53(9):1301-1314.
- Rigo RB, Panyella MG, Bartolomé LR, Ramos PA, Soria PR, Navarro MA. Variations observed for insulin concentrations in an interlaboratory quality control program may be due to interferences between reagents and the matrix of the control materials. Clin Biochem. 2007;40(13-14):1088-1091.
- Armbruster DA, Pry T. Limit of blank, limit of detection and limit of quantitation. Clin Biochem Rev. 2008;29 Suppl 1(Suppl 1):S49-52.
- Spencer C, LoPresti J, Fatemi S. How sensitive (second-generation) thyroglobulin measurement is changing paradigms for monitoring patients with differentiated thyroid cancer, in the absence or presence of thyroglobulin autoantibodies. Current opinion in endocrinology, diabetes, and obesity. 2014;21(5):394-404.
- Rawlins ML, Roberts WL. Performance characteristics of six third-generation assays for thyroid-stimulating hormone. Clin Chem. 2004;50(12):2338-2344.
- Schaaf L, Theodoropoulou M, Gregori A, Leiprecht A, Trojan J, Klostermeier J, Stalla GK. Thyrotropin-releasing hormone time-dependently influences thyrotropin microheterogeneity--an in vivo study in euthyroidism. J Endocrinol. 2000;166(1):137-143.
- Donadio S, Morelle W, Pascual A, Romi-Lebrun R, Michalski JC, Ronin C. Both core and terminal glycosylation alter epitope expression in thyrotropin and introduce discordances in hormone measurements. Clin Chem Lab Med. 2005;43(5):519-530.
- Estrada JM, Soldin D, Buckey TM, Burman KD, Soldin OP. Thyrotropin isoforms: implications for thyrotropin analysis and clinical practice. Thyroid. 2014;24(3):411-423.
- Persani L. Hypothalamic thyrotropin-releasing hormone and thyrotropin biological activity. Thyroid. 1998;8(10):941-946.
- Ikegami K, Liao XH, Hoshino Y, Ono H, Ota W, Ito Y, Nishiwaki-Ohkawa T, Sato C, Kitajima K, Iigo M, Shigeyoshi Y, Yamada M, Murata Y, Refetoff S, Yoshimura T. Tissue-specific posttranslational modification allows functional targeting of thyrotropin. Cell Rep. 2014;9(3):801-810.
- Persani L, C A, P B-P. Dissociation between immunological and buiological activities of circulating TSH. Exp Clin Endocrinol. 1994;102:38-48.
- Oliveira JH, Barbosa ER, Kasamatsu T, Abucham J. Evidence for thyroid hormone as a positive regulator of serum thyrotropin bioactivity. J Clin Endocrinol Metab. 2007;92(8):3108-3113.
- Yoshihara A, Noh JY, Watanabe N, Iwaku K, Kunii Y, Ohye H, Suzuki M, Matsumoto M, Suzuki N, Sugino K, Thienpont LM, Hishinuma A, Ito K. Seasonal Changes in Serum Thyrotropin Concentrations Observed from Big Data Obtained During Six Consecutive Years from 2010 to 2015 at a Single Hospital in Japan. Thyroid.2018;28(4):429-436.
- Persani L, Asteria C, Tonacchera M, Vitti P, Krishna V, Chatterjee K, Beck-Peccoz P. Evidence for the secretion of thyrotropin with enhanced bioactivity in syndromes of thyroid hormone resistance. J Clin Endocrinol Metab.1994;78:1035-1039.
- Persani L, Ferretti E, Borgato S, Faglia G, Beck-Peccoz P. Circulating thyrotropin bioactivity in sporadic central hypothyroidism. J Clin Endocrinol Metab. 2000;85(10):3631-3635.
- Pappa T, Johannesen J, Scherberg N, Torrent M, Dumitrescu A, Refetoff S. A TSHβ Variant with Impaired Immunoreactivity but Intact Biological Activity and Its Clinical Implications. Thyroid. 2015;25(8):869-876.
- Andersen S, Pedersen KM, Bruun NH, Laurberg P. Narrow individual variations in serum T4 and T3 in normal subjects: a clue to the understanding of subclinical thyroid disease. J Clin Endocrinol Metab. 2002;87:1068-1072.
- Boas M, Forman JL, Juul A, Feldt-Rasmussen U, Skakkebaek NE, Hilsted L, Chellakooty M, Larsen T, Larsen JF, Petersen JH, Main KM. Narrow intra-individual variation of maternal thyroid function in pregnancy based on a longitudinal study on 132 women. Eur J Endocrinol. 2009;161(6):903-910.
- Meikle AW, Stringham JD, Woodward MG, Nelson JC. Hereditary and environmental influences on the variation of thyroid hormones in normal male twins. J Clin Endocrinol Metab1. 1988;66:588-592.
- Hansen PS, Brix TH, Sørensen TI, Kyvik KO, Hegedüs L. Major genetic influence on the regulation of the pituitary-thyroid axis: a study of healthy Danish twins. J Clin Endocrinol Metab. 2004;89(3):1181-1187.
- Panicker V, Wilson SG, Spector TD, Brown SJ, Falchi M, Richards JB, Surdulescu GL, Lim EM, Fletcher SJ, Walsh JP. Heritability of serum TSH, free T4 and free T3 concentrations: a study of a large UK twin cohort. Clin Endocrinol (Oxf). 2008;68(4):652-659.
- Arnaud-Lopez L, Usala G, Ceresini G, Mitchell BD, Pilia MG, Piras MG, Sestu N, Maschio A, Busonero F, Albai G, Dei M, Lai S, Mulas A, Crisponi L, Tanaka T, Bandinelli S, Guralnik JM, Loi A, Balaci L, Sole G, Prinzis A, Mariotti S, Shuldiner AR, Cao A, Schlessinger D, Uda M, Abecasis GR, Nagaraja R, Sanna S, Naitza S. Phosphodiesterase 8B gene variants are associated with serum TSH levels and thyroid function. American journal of human genetics. 2008;82(6):1270-1280.
- Larsen CC, Karaviti LP, Seghers V, Weiss RE, Refetoff S, Dumitrescu AM. A new family with an activating mutation (G431S) in the TSH receptor gene: a phenotype discussion and review of the literature. Int J Pediatr Endocrinol. 2014;2014(1):23.
- De Marco G, Agretti P, Camilot M, Teofoli F, Tatò L, Vitti P, Pinchera A, Tonacchera M. Functional studies of new TSH receptor (TSHr) mutations identified in patients affected by hypothyroidism or isolated hyperthyrotrophinaemia. Clin Endocrinol (Oxf). 2009;70(2):335-338.
- Alberti L, Proverbio MC, Costagliola S, Romoli R, Boldrighini B, Vigone MC, Weber G, Chiumello G, Beck-Peccoz P, Persani L. Germline mutations of TSH receptor gene as cause of nonautoimmune subclinical hypothyroidism. J Clin Endocrinol Metab. 2002;87(6):2549-2555.
- van de Ven AC, Netea-Maier RT, Medici M, Sweep FC, Ross HA, Hofman A, de Graaf J, Kiemeney LA, Hermus AR, Peeters RP, Visser TJ, den Heijer M. Underestimation of effect of thyroid function parameters on morbidity and mortality due to intra-individual variation. J Clin Endocrinol Metab. 2011;96(12):E2014-2017.
- Jonklaas J. TSH Reference Intervals: Their Importance and Complexity. Thyroid. 2024;34(8):957-959.
- Kuś A, Sterenborg R, Haug EB, Galesloot TE, Visser WE, Smit JWA, Bednarczuk T, Peeters RP, Åsvold BO, Teumer A, Medici M. Towards Personalized TSH Reference Ranges: A Genetic and Population-Based Approach in Three Independent Cohorts. Thyroid. 2024;34(8):969-979.
- Biondi B, Cooper DS. The clinical significance of subclinical thyroid dysfunction. Endocr Rev. 2008;29(1):76-131.
- Coene KL, Demir AY, Broeren MA, Verschuure P, Lentjes EG, Boer AK. Subclinical hypothyroidism: a 'laboratory-induced' condition? Eur J Endocrinol. 2015;173(4):499-505.
- Stöckl D, Van Uytfanghe K, Van Aelst S, Thienpont LM. A statistical basis for harmonization of thyroid stimulating hormone immunoassays using a robust factor analysis model. Clin Chem Lab Med. 2014;52(7):965-972.
- Strich D, Karavani G, Levin S, Edri S, Gillis D. Normal limits for serum thyrotropin vary greatly depending on method. Clin Endocrinol (Oxf). 2016;85(1):110-115.
- Solberg HE. The IFCC recommendation on estimation of reference intervals. The RefVal program. Clin Chem Lab Med. 2004;42(7):710-714.
- Kahapola-Arachchige KM, Hadlow N, Wardrop R, Lim EM, Walsh JP. Age-specific TSH reference ranges have minimal impact on the diagnosis of thyroid dysfunction. Clin Endocrinol (Oxf). 2012;77(5):773-779.
- Fan L, Bu Y, Chen S, Wang S, Zhang W, He Y, Sun D. Iodine nutritional status and its associations with thyroid function of pregnant women and neonatal TSH. Front Endocrinol (Lausanne). 2024;15:1394306.
- Jensen E, Hyltoft Petersen P, Blaabjerg O, Hansen PS, Brix TH, Kyvik KO, Hegedüs L. Establishment of a serum thyroid stimulating hormone (TSH) reference interval in healthy adults. The importance of environmental factors, including thyroid antibodies. Clin Chem Lab Med. 2004;42(7):824-832.
- Spencer CA, Hollowell JG, Kazarosyan M, Braverman LE. National Health and Nutrition Examination Survey III thyroid-stimulating hormone (TSH)-thyroperoxidase antibody relationships demonstrate that TSH upper reference limits may be skewed by occult thyroid dysfunction. J Clin Endocrinol Metab. 2007;92(11):4236-4240.
- Surks MI, Hollowell JG. Age-specific distribution of serum thyrotropin and antithyroid antibodies in the US population: implications for the prevalence of subclinical hypothyroidism. J Clin Endocrinol Metab.2007;92(12):4575-4582.
- Surks MI, Boucai L. Age- and race-based serum thyrotropin reference limits. J Clin Endocrinol Metab.2010;95(2):496-502.
- Sletner L, Jenum AK, Qvigstad E, Hammerstad SS. Thyroid Function During Pregnancy in A Multiethnic Population in Norway. J Endocr Soc. 2021;5(7):bvab078.
- Vejbjerg P, Knudsen N, Perrild H, Carlé A, Laurberg P, Pedersen IB, Rasmussen LB, Ovesen L, Jørgensen T. The impact of smoking on thyroid volume and function in relation to a shift towards iodine sufficiency. Eur J Epidemiol.2008;23(6):423-429.
- Ittermann T, Khattak RM, Nauck M, Cordova CM, Völzke H. Shift of the TSH reference range with improved iodine supply in Northeast Germany. Eur J Endocrinol. 2015;172(3):261-267.
- Nyrnes A, Jorde R, Sundsfjord J. Serum TSH is positively associated with BMI. Int J Obes (Lond). 2006;30(1):100-105.
- Duntas LH, Biondi B. The interconnections between obesity, thyroid function, and autoimmunity: the multifold role of leptin. Thyroid. 2013;23(6):646-653.
- Soldin OP, Goughenour BE, Gilbert SZ, Landy HJ, Soldin SJ. Thyroid hormone levels associated with active and passive cigarette smoking. Thyroid. 2009;19(8):817-823.
- Raverot V, Bonjour M, Abeillon du Payrat J, Perrin P, Roucher-Boulez F, Lasolle H, Subtil F, Borson-Chazot F. Age- and Sex-Specific TSH Upper-Limit Reference Intervals in the General French Population: There Is a Need to Adjust Our Actual Practices. J Clin Med. 2020;9(3).
- Meisinger C, Ittermann T, Wallaschofski H, Heier M, Below H, Kramer A, Döring A, Nauck M, Völzke H. Geographic variations in the frequency of thyroid disorders and thyroid peroxidase antibodies in persons without former thyroid disease within Germany. Eur J Endocrinol. 2012;167(3):363-371.
- Berghout A, Wiersinga WM, Smits NJ, touber JL. Interrelationships between age, thyroid volume, thyroid nodularity, and thyroid function in patients with sporadic nontoxic goiter. Amer J Med. 1990;89:602-608.
- Atzmon G, Barzilai N, Hollowell JG, Surks MI, Gabriely I. Extreme longevity is associated with increased serum thyrotropin. J Clin Endocrinol Metab. 2009;94(4):1251-1254.
- Akirov A, Gimbel H, Grossman A, Shochat T, Shimon I. Elevated TSH in adults treated for hypothyroidism is associated with increased mortality. Eur J Endocrinol. 2017;176(1):57-66.
- Somwaru LL, Rariy CM, Arnold AM, Cappola AR. The natural history of subclinical hypothyroidism in the elderly: the cardiovascular health study. J Clin Endocrinol Metab. 2012;97(6):1962-1969.
- Kapelari K, Kirchlechner C, Högler W, Schweitzer K, Virgolini I, Moncayo R. Pediatric reference intervals for thyroid hormone levels from birth to adulthood: a retrospective study. BMC Endocr Disord. 2008;8:15.
- Verburg FA, Kirchgässner C, Hebestreit H, Steigerwald U, Lentjes EG, Ergezinger K, Grelle I, Reiners C, Luster M. Reference ranges for analytes of thyroid function in children. Horm Metab Res. 2011;43(6):422-426.
- Goichot B, Sapin R, Schlienger JL. Subclinical hyperthyroidism: considerations in defining the lower limit of the thyrotropin reference interval. Clin Chem. 2009;55(3):420-424.
- Biondi B, Cooper DS. Subclinical Hyperthyroidism. N Engl J Med. 2018;379(15):1485-1486.
- Kim HJ, McLeod DSA. Subclinical Hyperthyroidism and Cardiovascular Disease. Thyroid. 2024.
- Canaris GJ, Manowitz NR, Mayor G, Ridgway EC. The Colorado thyroid disease prevalence study. Arch Intern Med. 2000;160:526-534.
- Surks MI, Ortiz E, Daniels GH, Sawin CT, Col NF, Cobin RH, Franklyn JA, Hershman JM, Burman KD, Denke MA, Gorman C, Cooper RS, Weissman NJ. Subclinical thyroid disease: scientific review and guidelines for diagnosis and management. Jama. 2004;291(2):228-238.
- Efthymiadis A, Henry M, Spinos D, Bourlaki M, Tsikopoulos A, Bourazana A, Bastounis A, Tsikopoulos K. Adequacy of thyroid hormone replacement for people with hypothyroidism in real-world settings: A systematic review and meta-analysis of observational studies. Clin Endocrinol (Oxf). 2024;100(5):488-501.
- Yu OHY, Filliter C, Filion KB, Platt RW, Grad R, Renoux C. Levothyroxine Treatment of Subclinical Hypothyroidism and the Risk of Adverse Cardiovascular Events. Thyroid. 2024;34(10):1214-1224.
- Blum MR, Bauer DC, Collet TH, Fink HA, Cappola AR, da Costa BR, Wirth CD, Peeters RP, Åsvold BO, den Elzen WP, Luben RN, Imaizumi M, Bremner AP, Gogakos A, Eastell R, Kearney PM, Strotmeyer ES, Wallace ER, Hoff M, Ceresini G, Rivadeneira F, Uitterlinden AG, Stott DJ, Westendorp RG, Khaw KT, Langhammer A, Ferrucci L, Gussekloo J, Williams GR, Walsh JP, Jüni P, Aujesky D, Rodondi N. Subclinical thyroid dysfunction and fracture risk: a meta-analysis. Jama. 2015;313(20):2055-2065.
- Collet TH, Gussekloo J, Bauer DC, den Elzen WP, Cappola AR, Balmer P, Iervasi G, Åsvold BO, Sgarbi JA, Völzke H, Gencer B, Maciel RM, Molinaro S, Bremner A, Luben RN, Maisonneuve P, Cornuz J, Newman AB, Khaw KT, Westendorp RG, Franklyn JA, Vittinghoff E, Walsh JP, Rodondi N. Subclinical hyperthyroidism and the risk of coronary heart disease and mortality. Arch Intern Med. 2012;172(10):799-809.
- Taylor PN, Razvi S, Pearce SH, Dayan CM. Clinical review: A review of the clinical consequences of variation in thyroid function within the reference range. J Clin Endocrinol Metab. 2013;98(9):3562-3571.
- Jay M, Huan P, Cliffe N, Rakoff J, Morris E, Kavsak P, Luthra M, Punthakee Z. Treatment of Subclinical Hyperthyroidism and Incident Atrial Fibrillation. Clin Endocrinol (Oxf). 2024.
- Zhang X, Zhang G, Wang S, Jin J, Zhang S, Teng X. The change in thyroid function categories with time in patients with subclinical hypothyroidism: a systematic review and meta-analysis. BMC Endocr Disord.2024;24(1):224.
- McQuade C, Skugor M, Brennan DM, Hoar B, Stevenson C, Hoogwerf BJ. Hypothyroidism and moderate subclinical hypothyroidism are associated with increased all-cause mortality independent of coronary heart disease risk factors: a PreCIS database study. Thyroid. 2011;21(8):837-843.
- Javed Z, Sathyapalan T. Levothyroxine treatment of mild subclinical hypothyroidism: a review of potential risks and benefits. Ther Adv Endocrinol Metab. 2016;7(1):12-23.
- Helfand M. Screening for subclinical thyroid dysfunction in nonpregnant adults: a summary of the evidence for the U.S. Preventive Services Task Force. Ann Intern Med. 2004;140(2):128-141.
- Åsvold BO, Vatten LJ, Midthjell K, Bjøro T. Serum TSH within the reference range as a predictor of future hypothyroidism and hyperthyroidism: 11-year follow-up of the HUNT Study in Norway. J Clin Endocrinol Metab.2012;97(1):93-99.
- Åsvold BO, Vatten LJ, Bjøro T, Bauer DC, Bremner A, Cappola AR, Ceresini G, den Elzen WP, Ferrucci L, Franco OH, Franklyn JA, Gussekloo J, Iervasi G, Imaizumi M, Kearney PM, Khaw KT, Maciel RM, Newman AB, Peeters RP, Psaty BM, Razvi S, Sgarbi JA, Stott DJ, Trompet S, Vanderpump MP, Völzke H, Walsh JP, Westendorp RG, Rodondi N. Thyroid function within the normal range and risk of coronary heart disease: an individual participant data analysis of 14 cohorts. JAMA Intern Med. 2015;175(6):1037-1047.
- Asvold BO, Bjøro T, Vatten LJ. Association of thyroid function with estimated glomerular filtration rate in a population-based study: the HUNT study. Eur J Endocrinol. 2011;164(1):101-105.
- Cappola AR, Ladenson PW. Hypothyroidism and atherosclerosis. J Clin Endocrinol Metab. 2003;88(6):2438-2444.
- Ochs N, Auer R, Bauer DC, Nanchen D, Gussekloo J, Cornuz J, Rodondi N. Meta-analysis: subclinical thyroid dysfunction and the risk for coronary heart disease and mortality. Ann Intern Med. 2008;148(11):832-845.
- Ittermann T, Lorbeer R, Dörr M, Schneider T, Quadrat A, Heßelbarth L, Wenzel M, Lehmphul I, Köhrle J, Mensel B, Völzke H. High levels of thyroid-stimulating hormone are associated with aortic wall thickness in the general population. Eur Radiol. 2016;26(12):4490-4496.
- Andersen MN, Olsen AS, Madsen JC, Kristensen SL, Faber J, Torp-Pedersen C, Gislason GH, Selmer C. Long-Term Outcome in Levothyroxine Treated Patients With Subclinical Hypothyroidism and Concomitant Heart Disease. J Clin Endocrinol Metab. 2016;101(11):4170-4177.
- Baretella O, Blum MR, Abolhassani N, Alwan H, Wildisen L, Del Giovane C, Tal K, Moutzouri E, Åsvold BO, Cappola AR, Gussekloo J, Iacoviello M, Iervasi G, Imaizumi M, Weiler S, Razvi S, Sgarbi JA, Völzke H, Brown SJ, Walsh JP, Vaes B, Yeap BB, Dullaart RPF, Bakker SJL, Kavousi M, Ceresini G, Ferrucci L, Aujesky D, Peeters RP, Bauer DC, Feller M, Rodondi N. Associations between subclinical thyroid dysfunction and cardiovascular risk factors according to age and sex. J Clin Endocrinol Metab. 2024.
- Iqbal A, Jorde R, Figenschau Y. Serum lipid levels in relation to serum thyroid-stimulating hormone and the effect of thyroxine treatment on serum lipid levels in subjects with subclinical hypothyroidism: the Tromsø Study. J Intern Med. 2006;260(1):53-61.
- Asvold BO, Bjøro T, Vatten LJ. Associations of TSH levels within the reference range with future blood pressure and lipid concentrations: 11-year follow-up of the HUNT study. Eur J Endocrinol. 2013;169(1):73-82.
- Casey BM, Dashe JS, Wells CE, McIntire DD, Leveno KJ, Cunningham FG. Subclinical hyperthyroidism and pregnancy outcomes. Obstet Gynecol. 2006;107(2 Pt 1):337-341.
- Ajmani SN, Aggarwal D, Bhatia P, Sharma M, Sarabhai V, Paul M. Prevalence of overt and subclinical thyroid dysfunction among pregnant women and its effect on maternal and fetal outcome. J Obstet Gynaecol India.2014;64(2):105-110.
- Debbarma R, Gothwal M, Singh P, Yadav G, Purohit P, Ghuman NK, Gupta N. The Spectrum of Thyroid Dysfunction During Pregnancy and Fetomaternal Outcome, A Study from the Premier Institute of Western India. Indian J Community Med. 2024;49(5):734-738.
- Zaccarelli-Marino MA, Dsouki NA, de Carvalho RP, Maciel RMB. Evaluation of Anti-Thyroperoxidase (A-TPO) and Anti-Thyroglobulin (A-Tg) Antibodies in Women with Previous Hashimoto's Thyroiditis during and after Pregnancy. J Clin Med. 2024;13(15).
- Tong Z, Xiaowen Z, Baomin C, Aihua L, Yingying Z, Weiping T, Zhongyan S. The Effect of Subclinical Maternal Thyroid Dysfunction and Autoimmunity on Intrauterine Growth Restriction: A Systematic Review and Meta-Analysis. Medicine (Baltimore). 2016;95(19):e3677.
- Iijima S. Pitfalls in the assessment of gestational transient thyrotoxicosis. Gynecol Endocrinol. 2020;36(8):662-667.
- Brabant G, Peeters RP, Chan SY, Bernal J, Bouchard P, Salvatore D, Boelaert K, Laurberg P. Management of subclinical hypothyroidism in pregnancy: are we too simplistic? Eur J Endocrinol. 2015;173(1):P1-p11.
- Negro R, Stagnaro-Green A. Diagnosis and management of subclinical hypothyroidism in pregnancy. Bmj.2014;349:g4929.
- Negro R. Thyroid autoimmunity and pre-term delivery: brief review and meta-analysis. J Endocrinol Invest.2011;34(2):155-158.
- Negro R, Schwartz A, Stagnaro-Green A. Impact of Levothyroxine in Miscarriage and Preterm Delivery Rates in First Trimester Thyroid Antibody-Positive Women With TSH Less Than 2.5 mIU/L. J Clin Endocrinol Metab.2016;101(10):3685-3690.
- Chen X, Jin B, Xia J, Tao X, Huang X, Sun L, Yuan Q. Effects of Thyroid Peroxidase Antibody on Maternal and Neonatal Outcomes in Pregnant Women in an Iodine-Sufficient Area in China. Int J Endocrinol.2016;2016:6461380.
- Dhillon-Smith RK, Tobias A, Smith PP, Middleton LJ, Sunner KK, Baker K, Farrell-Carver S, Bender-Atik R, Agrawal R, Bhatia K, Chu JJ, Edi-Osagie E, Ewies A, Ghobara T, Gupta P, Jurkovic D, Khalaf Y, Mulbagal K, Nunes N, Overton C, Quenby S, Rai R, Raine-Fenning N, Robinson L, Ross J, Sizer A, Small R, Underwood M, Kilby MD, Daniels J, Thangaratinam S, Chan S, Boelaert K, Coomarasamy A. The Prevalence of Thyroid Dysfunction and Autoimmunity in Women With History of Miscarriage or Subfertility. J Clin Endocrinol Metab.2020;105(8).
- Yu M, Long Y, Wang Y, Zhang R, Tao L. Effect of levothyroxine on the pregnancy outcomes in recurrent pregnancy loss women with subclinical hypothyroidism and thyroperoxidase antibody positivity: a systematic review and meta-analysis. J Matern Fetal Neonatal Med. 2023;36(2):2233039.
- Maraka S, Dosiou C. Subclinical Hypothyroidism and Thyroid Autoimmunity in Pregnancy: To Treat or Not to Treat. Endocrinol Metab Clin North Am. 2024;53(3):363-376.
- Wilson KL, Casey BM, McIntire DD, Halvorson LM, Cunningham FG. Subclinical thyroid disease and the incidence of hypertension in pregnancy. Obstet Gynecol. 2012;119(2 Pt 1):315-320.
- Alavi A, Adabi K, Nekuie S, Jahromi EK, Solati M, Sobhani A, Karmostaji H, Jahanlou AS. Thyroid dysfunction and autoantibodies association with hypertensive disorders during pregnancy. J Pregnancy. 2012;2012:742695.
- Nazarpour S, Ramezani Tehrani F, Rahmati M, Azizi F. Prediction of preterm delivery based on thyroid peroxidase antibody levels and other identified risk factors. Eur J Obstet Gynecol Reprod Biol. 2023;284:125-130.
- Liu X, Zhang C, Lin Z, Zhu K, He R, Jiang Z, Wu H, Yu J, Luo Q, Sheng J, Fan J, Pan J, Huang H. Association of maternal mild hypothyroidism in the first and third trimesters with obstetric and perinatal outcomes: a prospective cohort study. Am J Obstet Gynecol. 2024.
- Haddow JE, Palomaki GE, Allan WC, Williams JR KG, Gagnon J, O'Heir CE, Mitchell ML, Hermos RJ WS, Faix JD, Klein RZ. Maternal thyroid deficiency during pregnancy and subsequent neuropsychological development of the child. NEJM. 1999;341:549-555.
- Li Y, Shan Z, Teng W, Yu X, Li Y, Fan C, Teng X, Guo R, Wang H, Li J, Chen Y, Wang W, Chawinga M, Zhang L, Yang L, Zhao Y, Hua T. Abnormalities of maternal thyroid function during pregnancy affect neuropsychological development of their children at 25-30 months. Clin Endocrinol (Oxf). 2010;72(6):825-829.
- Nazarpour S, Ramezani Tehrani F, Simbar M, Tohidi M, Alavi Majd H, Azizi F. Effects of levothyroxine treatment on pregnancy outcomes in pregnant women with autoimmune thyroid disease. Eur J Endocrinol. 2017;176(2):253-265.
- Pekonen F, Alfthan H, Stenman UH, Ylikorkala O. Human chorionic gonadotropin (hCG) and thyroid function in early human pregnancy: circadian variation and evidence for intrinsic thyrotropic activity of hCG. J Clin Endocrinol Metab. 1988;66(4):853-856.
- Goodwin TM, Montoro M, Mestman JH, Pekary AE, Hershman JM. The role of chorionic gonadotropin in transient hyperthyroidism of hyperemesis gravidarum. J Clin Endocrinol Metab. 1992;75(5):1333-1337.
- Korevaar TI, Steegers EA, de Rijke YB, Visser WE, Jaddoe VW, Visser TJ, Medici M, Peeters RP. Placental Angiogenic Factors Are Associated With Maternal Thyroid Function and Modify hCG-Mediated FT4 Stimulation. J Clin Endocrinol Metab. 2015;100(10):E1328-1334.
- Grün JP, Meuris S, De Nayer P, Glinoer D. The thyrotrophic role of human chorionic gonadotrophin (hCG) in the early stages of twin (versus single) pregnancies. Clin Endocrinol (Oxf). 1997;46(6):719-725.
- Barjaktarovic M, Korevaar TIM, Jaddoe VWV, de Rijke YB, Peeters RP, Steegers EAP. Human chorionic gonadotropin and risk of pre-eclampsia: prospective population-based cohort study. Ultrasound Obstet Gynecol.2019;54(4):477-483.
- Männistö T, Surcel HM, Ruokonen A, Vääräsmäki M, Pouta A, Bloigu A, Järvelin MR, Hartikainen AL, Suvanto E. Early pregnancy reference intervals of thyroid hormone concentrations in a thyroid antibody-negative pregnant population. Thyroid. 2011;21(3):291-298.
- McNeil AR, Stanford PE. Reporting Thyroid Function Tests in Pregnancy. Clin Biochem Rev. 2015;36(4):109-126.
- Tozzoli R, D'Aurizio F, Ferrari A, Castello R, Metus P, Caruso B, Perosa AR, Sirianni F, Stenner E, Steffan A, Villalta D. The upper reference limit for thyroid peroxidase autoantibodies is method-dependent: A collaborative study with biomedical industries. Clin Chim Acta. 2016;452:61-65.
- Caruso B, Bovo C, Guidi GC. Causes of Preanalytical Interferences on Laboratory Immunoassays - A Critical Review. Ejifcc. 2020;31(1):70-84.
- Roelfsema F, Pijl H, Kok P, Endert E, Fliers E, Biermasz NR, Pereira AM, Veldhuis JD. Thyrotropin secretion in healthy subjects is robust and independent of age and gender, and only weakly dependent on body mass index. J Clin Endocrinol Metab. 2014;99(2):570-578.
- Roelfsema F, Boelen A, Kalsbeek A, Fliers E. Regulatory aspects of the human hypothalamus-pituitary-thyroid axis. Best practice & research Clinical endocrinology & metabolism. 2017;31(5):487-503.
- van der Spoel E, Roelfsema F, van Heemst D. Within-Person Variation in Serum Thyrotropin Concentrations: Main Sources, Potential Underlying Biological Mechanisms, and Clinical Implications. Front Endocrinol (Lausanne).2021;12:619568.
- Beckett G, MacKenzie F. Thyroid guidelines - are thyroid-stimulating hormone assays fit for purpose? Ann Clin Biochem. 2007;44(Pt 3):203-208.
- Roelfsema F, Pereira AM, Veldhuis JD, Adriaanse R, Endert E, Fliers E, Romijn JA. Thyrotropin secretion profiles are not different in men and women. J Clin Endocrinol Metab. 2009;94(10):3964-3967.
- Walsh JP, Ward LC, Burke V, Bhagat CI, Shiels L, Henley D, Gillett MJ, Gilbert R, Tanner M, Stuckey BG. Small changes in thyroxine dosage do not produce measurable changes in hypothyroid symptoms, well-being, or quality of life: results of a double-blind, randomized clinical trial. J Clin Endocrinol Metab. 2006;91(7):2624-2630.
- Jonklaas J, Sarlis NJ, Litofsky D, Ain KB, Bigos ST, Brierley JD, Cooper DS, Haugen BR, Ladenson PW, Magner J, Robbins J, Ross DS, Skarulis M, Maxon HR, Sherman SI. Outcomes of patients with differentiated thyroid carcinoma following initial therapy. Thyroid. 2006;16(12):1229-1242.
- Cooper DS, Doherty GM, Haugen BR, Kloos RT, Lee SL, Mandel SJ, Mazzaferri EL, McIver B, Pacini F, Schlumberger M, Sherman SI, Steward DL, Tuttle RM. Revised American Thyroid Association management guidelines for patients with thyroid nodules and differentiated thyroid cancer. Thyroid. 2009;19(11):1167-1214.
- Mebis L, van den Berghe G. The hypothalamus-pituitary-thyroid axis in critical illness. Neth J Med.2009;67(10):332-340.
- Van den Berghe G. Non-thyroidal illness in the ICU: a syndrome with different faces. Thyroid. 2014;24(10):1456-1465.
- Aidoo ED, Ababio GK, Arko-Boham B, Tagoe EA, Aryee NA. Thyroid dysfunction among patients assessed by thyroid function tests at a tertiary care hospital: a retrospective study. Pan Afr Med J. 2024;49:7.
- Stockigt JR. Guidelines for diagnosis and monitoring of thyroid disease: nonthyroidal illness. Clin Chem.1996;42:188-192.
- Stathatos N, Levetan C, Burman KD, Wartofsky L. The controversy of the treatment of critically ill patients with thyroid hormone. Best practice & research Clinical endocrinology & metabolism. 2001;15(4):465-478.
- Bunevicius R, Steibliene V, Prange AJ, Jr. Thyroid axis function after in-patient treatment of acute psychosis with antipsychotics: a naturalistic study. BMC Psychiatry. 2014;14:279.
- Soh SB, Aw TC. Laboratory Testing in Thyroid Conditions - Pitfalls and Clinical Utility. Ann Lab Med. 2019;39(1):3-14.
- Uy HL, Reasner CA, Samuels MH. Pattern of recovery of the hypothalamic-pituitary-thyroid axis following radioactive iodine therapy in patients with Graves' disease. Am J Med. 1995;99(2):173-179.
- Persani L. Clinical review: Central hypothyroidism: pathogenic, diagnostic, and therapeutic challenges. J Clin Endocrinol Metab. 2012;97:3068-3078.
- Lania A, Persani L, Beck-Peccoz P. Central hypothyroidism. Pituitary. 2008;11(2):181-186.
- Roelfsema F, Kok S, Kok P, Pereira AM, Biermasz NR, Smit JW, Frolich M, Keenan DM, Veldhuis JD, Romijn JA. Pituitary-hormone secretion by thyrotropinomas. Pituitary. 2009;12(3):200-210.
- Beck-Peccoz P, Giavoli C, Lania A. A 2019 update on TSH-secreting pituitary adenomas. J Endocrinol Invest.2019;42(12):1401-1406.
- Refetoff S, Weiss RE, Usala SJ. The syndromes of resistance to thyroid hormone. Endocrine Rev. 1993;14:348-399.
- Persani L, Rodien P, Moran C, Edward Visser W, Groeneweg S, Peeters R, Refetoff S, Gurnell M, Beck-Peccoz P, Chatterjee K. 2024 European Thyroid Association Guidelines on diagnosis and management of genetic disorders of thyroid hormone transport, metabolism and action. Eur Thyroid J. 2024;13(4).
- Dumitrescu AM, Refetoff S. The syndromes of reduced sensitivity to thyroid hormone. Biochim Biophys Acta.2013;1830(7):3987-4003.
- Bertalan R, Sallai A, Sólyom J, Lotz G, Szabó I, Kovács B, Szabó E, Patócs A, Rácz K. Hyperthyroidism caused by a germline activating mutation of the thyrotropin receptor gene: difficulties in diagnosis and therapy. Thyroid.2010;20(3):327-332.
- Drees JC, Stone JA, Reamer CR, Arboleda VE, Huang K, Hrynkow J, Greene DN, Petrie MS, Hoke C, Lorey TS, Dlott RS. Falsely undetectable TSH in a cohort of South Asian euthyroid patients. J Clin Endocrinol Metab.2014;99(4):1171-1179.
- Kalveram L, Kleinau G, Szymańska K, Scheerer P, Rivero-Müller A, Grüters-Kieslich A, Biebermann H. The Pathogenic TSH β-subunit Variant C105Vfs114X Causes a Modified Signaling Profile at TSHR. Int J Mol Sci.2019;20(22).
- Emerson JF, Ngo G, Emerson SS. Screening for interference in immunoassays. Clin Chem. 2003;49:1163-1169.
- Nayeemuddin SN, Panigrahi A, Bhattacharjee R, Chowdhury S. Heterophilic Interference of Rheumatoid Factor in TSH Immunometric Assay: A Cross-Sectional Observational Study. Indian J Endocrinol Metab. 2024;28(1):29-34.
- Czernichow P, Vandalem JL, Hennen G. Transient neonatal hyperthyrotropinemia: a factitious syndrome due to the presence of heterophilic antibodies in the plasma of infants and their mothers. J Clin Endocrinol Metab.1981;53(2):387-393.
- Favresse J, Paridaens H, Pirson N, Maiter D, Gruson D. Massive interference in free T4 and free T3 assays misleading clinical judgment. Clin Chem Lab Med. 2017;55(4):e84-e86.
- Ohba K, Noh JY, Unno T, Satoh T, Iwahara K, Matsushita A, Sasaki S, Oki Y, Nakamura H. Falsely elevated thyroid hormone levels caused by anti-ruthenium interference in the Elecsys assay resembling the syndrome of inappropriate secretion of thyrotropin. Endocr J. 2012;59(8):663-667.
- Takahashi S, Nishikawa M, Nishihara E, Deguchi H, Kohsaka K, Yamaoka H, Hisakado M, Fukata S, Ito M, Miyauchi A, Akamizu T. Interference against a newly labeled substance with ruthenium sulfonate complexes showing discrepant thyroid function test results. Clin Chim Acta. 2024;553:117706.
- Elston MS, Sehgal S, Du Toit S, Yarndley T, Conaglen JV. Factitious Graves' Disease Due to Biotin Immunoassay Interference-A Case and Review of the Literature. J Clin Endocrinol Metab. 2016;101(9):3251-3255.
- Balzer AHA, Whitehurst CB. An Analysis of the Biotin-(Strept)avidin System in Immunoassays: Interference and Mitigation Strategies. Curr Issues Mol Biol. 2023;45(11):8733-8754.
- Lazarus JH, John R, Ginsberg J, Hughes IA, Shewring G, Smith BR, Woodhead JS, Hall R. Transient neonatal hyperthyrotrophinaemia: a serum abnormality due to transplacentally acquired antibody to thyroid stimulating hormone. Br Med J (Clin Res Ed). 1983;286(6365):592-594.
- Sazonova DV, Perepelova MA, Shutova AS, Nikankina LV, Kolesnikova GS, Pigarova EA, Dzeranova LK. [Combination of macro-TSH and macroprolactinemia phenomena in a patient with autoimmune thyroiditis and vitiligo]. Probl Endokrinol (Mosk). 2024;70(5):34-39.
- Hattori N, Ishihara T, Shimatsu A. Variability in the detection of macro TSH in different immunoassay systems. Eur J Endocrinol. 2016;174(1):9-15.
- Verhoye E, Van den Bruel A, Delanghe JR, Debruyne E, Langlois MR. Spuriously high thyrotropin values due to anti-thyrotropin antibodies in adult patients. Clin Chem Lab Med. 2009;47(5):604-606.
- Piticchio T, Chiardi I, Tumminia A, Frasca F, Rotondi M, Trimboli P. PEG Precipitation to Detect Macro-TSH in Clinical Practice: A Systematic Review. Clin Endocrinol (Oxf). 2024.
- Grünert SC, Schmidts M, Pohlenz J, Kopp MV, Uhl M, Schwab KO. Congenital Central Hypothyroidism due to a Homozygous Mutation in the TSHβ Subunit Gene. Case Rep Pediatr. 2011;2011:369871.
- Yeste D, Baz-Redón N, Antolín M, Garcia-Arumí E, Mogas E, Campos-Martorell A, González-Llorens N, Aguilar-Riera C, Soler-Colomer L, Clemente M, Fernández-Cancio M, Camats-Tarruella N. Genetic and Functional Studies of Patients with Thyroid Dyshormonogenesis and Defects in the TSH Receptor (TSHR). Int J Mol Sci. 2024;25(18).
- Feldt-Rasmussen U. Analytical and clinical performance goals for thyroid testing: thyroid antibodies. Clin Chem.1996;46:In press.
- Saravanan P, Dayan CM. Thyroid autoantibodies. Endocrinol Metab Clin North Am. 2001;30(2):315-337, viii.
- McLachlan SM, Rapoport B. Discoveries in Thyroid Autoimmunity in the Past Century. Thyroid. 2023;33(3):278-286.
- Massart C, Sapin R, Gibassier J, Agin A, d'Herbomez M. Intermethod variability in TSH-receptor antibody measurement: implication for the diagnosis of Graves disease and for the follow-up of Graves ophthalmopathy. Clin Chem. 2009;55(1):183-186.
- D'Aurizio F, Metus P, Ferrari A, Caruso B, Castello R, Villalta D, Steffan A, Gaspardo K, Pesente F, Bizzaro N, Tonutti E, Valverde S, Cosma C, Plebani M, Tozzoli R. Definition of the upper reference limit for thyroglobulin antibodies according to the National Academy of Clinical Biochemistry guidelines: comparison of eleven different automated methods. Auto Immun Highlights. 2017;8(1):8.
- Tozzoli R, Bagnasco M, Giavarina D, Bizzaro N. TSH receptor autoantibody immunoassay in patients with Graves' disease: improvement of diagnostic accuracy over different generations of methods. Systematic review and meta-analysis. Autoimmun Rev. 2012;12(2):107-113.
- Rapoport B, Chazenbalk GD, Jaume JC, McLachlan SM. The thyrotropin (TSH) receptor: interaction with TSH and autoantibodies. Endocr Rev. 1998;19(6):673-716.
- Davies T, Marians R, Latif R. The TSH receptor reveals itself. J Clin Invest. 2002;110(2):161-164.
- Barbesino G, Tomer Y. Clinical review: Clinical utility of TSH receptor antibodies. J Clin Endocrinol Metab.2013;98(6):2247-2255.
- Rees Smith B, McLachlan SM, Furmaniak J. Autoantibodies to the thyrotropin receptor. Endocr Rev.1988;9(1):106-121.
- Adams DD. Long-acting thyroid stimulator: how receptor autoimmunity was discovered. Autoimmunity.1988;1(1):3-9.
- McKenzie MJ, Zakarija M. Antibodies in autoimmune thyroid disease. 6th. Edition ed. Philadelphia: J B Lippincott.
- Ando T, Latif R, Davies TF. Thyrotropin receptor antibodies: new insights into their actions and clinical relevance. Best practice & research Clinical endocrinology & metabolism. 2005;19(1):33-52.
- Noh JY, Hamada N, Inoue Y, Abe Y, Ito K, Ito K. Thyroid-stimulating antibody is related to Graves' ophthalmopathy, but thyrotropin-binding inhibitor immunoglobulin is related to hyperthyroidism in patients with Graves' disease. Thyroid. 2000;10(9):809-813.
- Mizutori Y, Chen CR, Latrofa F, McLachlan SM, Rapoport B. Evidence that shed thyrotropin receptor A subunits drive affinity maturation of autoantibodies causing Graves' disease. J Clin Endocrinol Metab. 2009;94(3):927-935.
- Ko J, Kook KH, Yoon JS, Woo KI, Yang JW. Longitudinal association of thyroid-stimulating immunoglobulin levels with clinical characteristics in thyroid eye disease. BMJ Open. 2022;12(6):e050337.
- Abeillon-du Payrat J, Caron P. The key data from the 2022 European Thyroid Association congress: Toward personalized treatment of Graves' orbitopathy. Ann Endocrinol (Paris). 2023;84(6):756-757.
- Gupta MK. Thyrotropin-receptor antibodies in thyroid diseases: advances in detection techniques and clinical application. Clin Chem Acta. 2000;293:1-29.
- Michalek K, Morshed SA, Latif R, Davies TF. TSH receptor autoantibodies. Autoimmun Rev. 2009;9(2):113-116.
- Kahaly GJ. Bioassays for TSH Receptor Antibodies: Quo Vadis? Eur Thyroid J. 2015;4(1):3-5.
- Kahaly GJ, Diana T, Glang J, Kanitz M, Pitz S, König J. Thyroid Stimulating Antibodies Are Highly Prevalent in Hashimoto's Thyroiditis and Associated Orbitopathy. J Clin Endocrinol Metab. 2016;101(5):1998-2004.
- Morgenthaler NG, Ho SC, Minich WB. Stimulating and blocking thyroid-stimulating hormone (TSH) receptor autoantibodies from patients with Graves' disease and autoimmune hypothyroidism have very similar concentration, TSH receptor affinity, and binding sites. J Clin Endocrinol Metab. 2007;92(3):1058-1065.
- Yoshida K, Aizawa Y, Kaise N, Fukazawa H, Kiso Y, Sayama N, Mori K, Hori H, Abe K. Relationship between thyroid-stimulating antibodies and thyrotropin-binding inhibitory immunoglobulins years after administration of radioiodine for Graves' disease: retrospective clinical survey. J Endocrinol Invest. 1996;19(10):682-686.
- Quadbeck B, Hoermann R, Hahn S, Roggenbuck U, Mann K, Janssen OE. Binding, stimulating and blocking TSH receptor antibodies to the thyrotropin receptor as predictors of relapse of Graves' disease after withdrawal of antithyroid treatment. Horm Metab Res. 2005;37(12):745-750.
- McLachlan SM, Rapoport B. Thyrotropin-blocking autoantibodies and thyroid-stimulating autoantibodies: potential mechanisms involved in the pendulum swinging from hypothyroidism to hyperthyroidism or vice versa. Thyroid.2013;23(1):14-24.
- Kamijo K. Shift in Dominance from Blocking to Stimulating Type of Thyrotropin Receptor Antibodies, Resulting in Conversion from Hypothyroidism to Hyperthyroidism during Late Pregnancy. Intern Med. 2024;63(4):521-526.
- Seetharamaiah GS, Kurosky A, Desai RK, Dallas JS, Prabhakar BS. A recombinant extracellular domain of the thyrotropin (TSH) receptor binds TSH in the absence of membranes. Endocrinology. 1994;134(2):549-554.
- Kung AW, Jones BM. A change from stimulatory to blocking antibody activity in Graves' disease during pregnancy. J Clin Endocrinol Metab. 1998;83(2):514-518.
- Davies TF, Ando T, Lin RY, Tomer Y, Latif R. Thyrotropin receptor-associated diseases: from adenomata to Graves disease. J Clin Invest. 2005;115(8):1972-1983.
- Morshed SA, Ando T, Latif R, Davies TF. Neutral antibodies to the TSH receptor are present in Graves' disease and regulate selective signaling cascades. Endocrinology. 2010;151(11):5537-5549.
- Kamijo K. TSH-receptor antibodies determined by the first, second and third generation assays and thyroid-stimulating antibody in pregnant patients with Graves' disease. Endocr J. 2007;54(4):619-624.
- Ajjan RA, Weetman AP. Techniques to quantify TSH receptor antibodies. Nature clinical practice Endocrinology & metabolism. 2008;4(8):461-468.
- Diana T, Wüster C, Kanitz M, Kahaly GJ. Highly variable sensitivity of five binding and two bio-assays for TSH-receptor antibodies. J Endocrinol Invest. 2016;39(10):1159-1165.
- Zöphel K, Roggenbuck D, Schott M. Clinical review about TRAb assay's history. Autoimmun Rev. 2010;9(10):695-700.
- Iida Y, Konishi J, Kasagi K, Kuma K, Torizuka K. Detection of TSH-binding inhibitor immunoglobulins by using the triton-solubilized receptor from human thyroid membranes. Endocrinol Jpn. 1982;29(2):227-231.
- Ehlers M, Allelein S, Schott M. TSH-receptor autoantibodies: pathophysiology, assay methods, and clinical applications. Minerva Endocrinol. 2018;43(3):323-332.
- Massart C, Gibassier J, d'Herbomez M. Clinical value of M22-based assays for TSH-receptor antibody (TRAb) in the follow-up of antithyroid drug treated Graves' disease: comparison with the second generation human TRAb assay. Clin Chim Acta. 2009;407(1-2):62-66.
- Smith BR, Sanders J, Furmaniak J. TSH receptor antibodies. Thyroid. 2007;17(10):923-938.
- Feldt-Rasmussen U, Schleusener H, Carayon P. Meta-analysis evaluation of the impact of thyrotropin receptor antibodies on long term remission after medical therapy of Graves' disease. J Clin Endocrinol Metab.1994;78(1):98-102.
- Furmaniak J, Sanders J, Sanders P, Miller-Gallacher J, Ryder MM, Rees Smith B. Practical applications of studies on the TSH receptor and TSH receptor autoantibodies. Endocrine. 2020;68(2):261-264.
- Evans M, Sanders J, Tagami T, Sanders P, Young S, Roberts E, Wilmot J, Hu X, Kabelis K, Clark J, Holl S, Richards T, Collyer A, Furmaniak J, Smith BR. Monoclonal autoantibodies to the TSH receptor, one with stimulating activity and one with blocking activity, obtained from the same blood sample. Clin Endocrinol (Oxf).2010;73(3):404-412.
- Takamura Y, Nakano K, Uruno T, Ito Y, Miya A, Kobayashi K, Yokozawa T, Matsuzuka F, Kuma K, Miyauchi A. Changes in serum TSH receptor antibody (TRAb) values in patients with Graves' disease after total or subtotal thyroidectomy. Endocr J. 2003;50(5):595-601.
- Carella C, Mazziotti G, Sorvillo F, Piscopo M, Cioffi M, Pilla P, Nersita R, Iorio S, Amato G, Braverman LE, Roti E. Serum thyrotropin receptor antibodies concentrations in patients with Graves' disease before, at the end of methimazole treatment, and after drug withdrawal: evidence that the activity of thyrotropin receptor antibody and/or thyroid response modify during the observation period. Thyroid. 2006;16(3):295-302.
- Giuliani C, Cerrone D, Harii N, Thornton M, Kohn LD, Dagia NM, Bucci I, Carpentieri M, Di Nenno B, Di Blasio A, Vitti P, Monaco F, Napolitano G. A TSHR-LH/CGR chimera that measures functional thyroid-stimulating autoantibodies (TSAb) can predict remission or recurrence in Graves' patients undergoing antithyroid drug (ATD) treatment. J Clin Endocrinol Metab. 2012;97(7):E1080-1087.
- McKenzie JM, Zakarija M. Fetal and neonatal hyperthyroidism and hypothyroidism due to maternal TSH receptor antibodies. Thyroid. 1992;2(2):155-159.
- Nor Azlin MI, Bakin YD, Mustafa N, Wahab NA, Johari MJ, Kamarudin NA, Jamil MA. Thyroid autoantibodies and associated complications during pregnancy. J Obstet Gynaecol. 2010;30(7):675-678.
- Hamada N, Momotani N, Ishikawa N, Yoshimura Noh J, Okamoto Y, Konishi T, Ito K, Ito K. Persistent high TRAb values during pregnancy predict increased risk of neonatal hyperthyroidism following radioiodine therapy for refractory hyperthyroidism. Endocr J. 2011;58(1):55-58.
- Heithorn R, Hauffa BP, Reinwein D. Thyroid antibodies in children of mothers with autoimmune thyroid disorders. Eur J Pediatr. 1999;158:24-28.
- Abeillon-du Payrat J, Chikh K, Bossard N, Bretones P, Gaucherand P, Claris O, Charrié A, Raverot V, Orgiazzi J, Borson-Chazot F, Bournaud C. Predictive value of maternal second-generation thyroid-binding inhibitory immunoglobulin assay for neonatal autoimmune hyperthyroidism. Eur J Endocrinol. 2014;171(4):451-460.
- Tokuda Y, Kasagi K, Iida Y, Hatabu H, Hidaka A, Misaki T, Konishi J. Sensitive, practical bioassay of thyrotropin, with use of FRTL-5 thyroid cells and magnetizable solid-phase-bound antibodies. Clin Chem. 1988;34(11):2360-2364.
- Morris JC, 3rd, Hay ID, Nelson RE, Jiang NS. Clinical utility of thyrotropin-receptor antibody assays: comparison of radioreceptor and bioassay methods. Mayo Clin Proc. 1988;63(7):707-717.
- Michelangeli VP, Munro DS, Poon CW, Frauman AG, Colman PG. Measurement of thyroid stimulating immunoglobulins in a new cell line transfected with a functional human TSH receptor (JPO9 cells), compared with an assay using FRTL-5 cells. Clin Endocrinol (Oxf). 1994;40(5):645-652.
- Michelangeli VP, Poon CW, Arnus EE, Frauman AG, Connelly J, Colman PG. Measurement of TSH receptor blocking immunoglobulins using 3H-adenine incorporation into FRTL-5 and JPO9 cells: use in a child with neonatal hypothyroidism. Clin Endocrinol (Oxf). 1995;42(1):39-44.
- Morgenthaler NG, Pampel I, Aust G, Seissler J, Scherbaum WA. Application of a bioassay with CHO cells for the routine detection of stimulating and blocking autoantibodies to the TSH-receptor. Horm Metab Res.1998;30(3):162-168.
- McLachlan SM, Rapoport B. Thyroid peroxidase autoantibody epitopes revisited*. Clin Endocrinol (Oxf).2008;69(4):526-527.
- Paparodis R, Livadas S, Karvounis E, Bantouna D, Zoupas I, Angelopoulos N, Imam S, Jaume JC. Elevated Preoperative TPO Ab Titers Decrease Risk for DTC in a Linear Fashion: A Retrospective Analysis of 1635 Cases. J Clin Endocrinol Metab. 2023;109(1):e347-e355.
- Jaume JC, Burek CL, Hoffman WH, Rose NR, McLachlan SM, Rapoport B. Thyroid peroxidase autoantibody epitopic 'fingerprints' in juvenile Hashimoto's thyroiditis: evidence for conservation over time and in families. Clin Exp Immunol. 1996;104(1):115-123.
- Ehlers M, Thiel A, Bernecker C, Porwol D, Papewalis C, Willenberg HS, Schinner S, Hautzel H, Scherbaum WA, Schott M. Evidence of a combined cytotoxic thyroglobulin and thyroperoxidase epitope-specific cellular immunity in Hashimoto's thyroiditis. J Clin Endocrinol Metab. 2012;97(4):1347-1354.
- Cayzer I, Chalmers SR, Doniach D, Swana G. An evaluation of two new haemagglutination tests for the rapid diagnosis of autoimmune thyroid diseases. J Clin Path. 1978;31:1147-1151.
- Mariotti S, Caturegli P, Piccolo P, Barbesino G, Pinchera A. Antithyroid peroxidase autoantibodies in thyroid diseases. J Clin Endocrinol Metab. 1990;71:661-669.
- Beever K, Bradbury J, Phillips D, McLachlan SM, Pegg C, Goral A, Overbeck W, Feifel G, Smith BR. Highly sensitive assays of autoantibodies to thyroglobulin and to thyroid peroxidase. Clin Chem. 1989;35:1949-1954.
- Laurberg P, Pedersen KM, Vittinghus E, Ekelund S. Sensitive enzyme-linked immunosorbent assay for measurement of autoantibodies to human thyroid peroxidase. Scandinavian Journal Of Clinical And Laboratory Investigation. 1992;52(7):663-669.
- La'ulu SL, Slev PR, Roberts WL. Performance characteristics of 5 automated thyroglobulin autoantibody and thyroid peroxidase autoantibody assays. Clin Chim Acta. 2007;376(1-2):88-95.
- Kasagi K, Takahashi N, Inoue G, Honda T, Kawachi Y, Izumi Y. Thyroid function in Japanese adults as assessed by a general health checkup system in relation with thyroid-related antibodies and other clinical parameters. Thyroid. 2009;19(9):937-944.
- Liu Q, Yang H, Chen Y, He X, Dong L, Zhang X, Yang Y, Tian M, Cheng W, Liu D, Yang G, Li K. Effect of thyroid peroxidase antibody titers trajectories during pregnancy and postpartum on postpartum thyroid dysfunction. Arch Gynecol Obstet. 2024.
- Karanikas G, Schuetz M, Wahl K, Paul M, Kontur S, Pietschmann P, Kletter K, Dudczak R, Willheim M. Relation of anti-TPO autoantibody titre and T-lymphocyte cytokine production patterns in Hashimoto's thyroiditis. Clin Endocrinol (Oxf). 2005;63(2):191-196.
- Rebuffat SA, Nguyen B, Robert B, Castex F, Peraldi-Roux S. Antithyroperoxidase antibody-dependent cytotoxicity in autoimmune thyroid disease. J Clin Endocrinol Metab. 2008;93(3):929-934.
- Carmel R, Spencer CA. Clinical and subclinical thyroid disorders associated with pernicious anemia. Arch Intern Med. 1982;142:1465-1469.
- Vanderpump MPJ, Tunbridge WMG, French JM, Appleton D, Bates D, Rodgers H, Evans JG, Clark F, Tunbridge F, Young ET. The incidence of thyroid disorders in the community; a twenty year follow up of the Whickham survey. Clin Endocrinol. 1995;43:55-68.
- Hutfless S, Matos P, Talor MV, Caturegli P, Rose NR. Significance of prediagnostic thyroid antibodies in women with autoimmune thyroid disease. J Clin Endocrinol Metab. 2011;96(9):E1466-1471.
- Yang S, Huang Z, Zhang Y, Li Y, Zhou Y, Guan H, Fan J. Association of Maternal Thyroglobulin Antibody with Preterm Birth in Euthyroid Women. J Clin Endocrinol Metab. 2025.
- Huber G, Staub JJ, Meier C, Mitrache C, Guglielmetti M, Huber P, Braverman LE. Prospective Study of the Spontaneous Course of Subclinical Hypothyroidism: Prognostic Value of Thyrotropin, Thyroid Reserve, and Thyroid Antibodies. J Clin Endocrinol Metab. 2002;87:3221-3226.
- Pedersen OM, Aardal NP, Larssen TB, Varhaug JE, Myking O, Vik-Mo H. The value of ultrasonography in predicting autoimmune thyroid disease. Thyroid. 2000;10:251-259.
- Mariotti S, Barbesino G, Caturegli P, Atzeni F, Manetti L, Marinò M, Grasso L, Velluzzi F, Loviselli A, Pinchera A, et al. False negative results observed in anti-thyroid peroxidase autoantibody determination by competitive radioimmunoassays using monoclonal antibodies. Eur J Endocrinol. 1994;130(6):552-558.
- Schmidt M, Voell M, Rahlff I, Dietlein M, Kobe C, Faust M, Schicha H. Long-term follow-up of antithyroid peroxidase antibodies in patients with chronic autoimmune thyroiditis (Hashimoto's thyroiditis) treated with levothyroxine. Thyroid. 2008;18(7):755-760.
- Bell TM, Bansal AS, Shorthouse C, Sandford N, Powell EE. Low titre autoantibodies predict autoimmune disease during interferon alpha treatment of chronic hepatitis C. J Gastroenterol Hepatol. 1999;14:419-422.
- Johnston AM, Eagles JM. Lithium-associated clinical hypothyroidism. Prevalence and risk factors. Br J Psychiatry.1999;175:336-339.
- Daniels GH. Amiodarone-induced thyrotoxicosis. J Clin Endocrinol Metab. 2001;86(1):3-8.
- Bocchetta A, Cocco F, Velluzzi F, Del Zompo M, Mariotti S, Loviselli A. Fifteen-year follow-up of thyroid function in lithium patients. J Endocrinol Invest. 2007;30(5):363-366.
- Tsang W, Houlden RL. Amiodarone-induced thyrotoxicosis: a review. Can J Cardiol. 2009;25(7):421-424.
- Wang L, Li B, Zhao H, Wu P, Wu Q, Chen K, Mu Y. A systematic review and meta-analysis of endocrine-related adverse events associated with interferon. Front Endocrinol (Lausanne). 2022;13:949003.
- Negro R, Žarković M, Attanasio R, Hegedüs L, Nagy EV, Papini E, Akarsu E, Alevizaki M, Ayvaz G, Bednarczuk T, Beleslin BN, Berta E, Bodor M, Borissova AM, Boyanov M, Buffet C, Burlacu MC, Ćirić J, Cohen CA, Díez JJ, Dobnig H, Fadeyev V, Field BCT, Fliers E, Führer D, Galofré JC, Hakala T, Jan J, Kopp P, Krebs M, Kršek M, Kužma M, Leenhardt L, Luchytskiy V, Puga FM, McGowan A, Melo M, Metso S, Moran C, Morgunova T, Niculescu DA, Perić B, Planck T, Poiana C, Robenshtok E, Rosselet PO, Ruchala M, Riis KR, Shepelkevich A, Tronko M, Unuane D, Vardarli I, Visser E, Vryonidou A, Younes YR, Perros P. Use of levothyroxine for euthyroid, thyroid antibody positive women with infertility: Analyses of aggregate data from a survey of European thyroid specialists (Treatment of Hypothyroidism in Europe by Specialists: An International Survey). Clin Endocrinol (Oxf).2024;101(2):180-190.
- Mecacci F, Parretti E, Cioni R, Lucchetti R, Magrini A, La Torre P, Mignosa M, Acanfora L, Mello G. Thyroid autoimmunity and its association with non-organ-specific antibodies and subclinical alterations of thyroid function in women with a history of pregnancy loss or preeclampsia. J Reprod Immunol. 2000;46:39-50.
- Bussen S, Steck T, Dietl J. Increased prevalence of thyroid antibodies in euthyroid women with a history of recurrent in-vitro fertilization failure. Hum Reprod. 2000;15:545-548.
- Poppe K, Glinoer D, tournaye H, Devroey P, Van Steirteghem a, Kaufman L, Velkeniers B. Assisted reproduction and thyroid autoimmunity: an unfortunate combination? J Clin Endocrinol Metab. 2003;88:4149-4152.
- Negro R, Formoso G, Coppola L, Presicce G, Mangieri T, Pezzarossa A, Dazzi D. Euthyroid women with autoimmune disease undergoing assisted reproduction technologies: the role of autoimmunity and thyroid function. J Endocrinol Invest. 2007;30(1):3-8.
- He X, Wang P, Wang Z, He X, Xu D, Wang B. Thyroid antibodies and risk of preterm delivery: a meta-analysis of prospective cohort studies. Eur J Endocrinol. 2012;167(4):455-464.
- Karakosta P, Alegakis D, Georgiou V, Roumeliotaki T, Fthenou E, Vassilaki M, Boumpas D, Castanas E, Kogevinas M, Chatzi L. Thyroid dysfunction and autoantibodies in early pregnancy are associated with increased risk of gestational diabetes and adverse birth outcomes. J Clin Endocrinol Metab. 2012;97(12):4464-4472.
- Bucci I, Giuliani C, Di Dalmazi G, Formoso G, Napolitano G. Thyroid Autoimmunity in Female Infertility and Assisted Reproductive Technology Outcome. Front Endocrinol (Lausanne). 2022;13:768363.
- McLachlan SM, Rapoport B. Why measure thyroglobulin autoantibodies rather than thyroid peroxidase autoantibodies? Thyroid. 2004;14(7):510-520.
- Latrofa F, Ricci D, Montanelli L, Rocchi R, Piaggi P, Sisti E, Grasso L, Basolo F, Ugolini C, Pinchera A, Vitti P. Thyroglobulin autoantibodies in patients with papillary thyroid carcinoma: comparison of different assays and evaluation of causes of discrepancies. J Clin Endocrinol Metab. 2012;97(11):3974-3982.
- Pickett AJ, Jones M, Evans C. Causes of discordance between thyroglobulin antibody assays. Ann Clin Biochem.2012;49(Pt 5):463-467.
- Spencer CA, Bergoglio LM, Kazarosyan M, Fatemi S, LoPresti JS. Clinical impact of thyroglobulin (Tg) and Tg autoantibody method differences on the management of patients with differentiated thyroid carcinomas. J Clin Endocrinol Metab. 2005;90(10):5566-5575.
- Lupoli GA, Okosieme OE, Evans C, Clark PM, Pickett AJ, Premawardhana LD, Lupoli G, Lazarus JH. Prognostic significance of thyroglobulin antibody epitopes in differentiated thyroid cancer. J Clin Endocrinol Metab.2015;100(1):100-108.
- Taylor KP, Parkington D, Bradbury S, Simpson HL, Jefferies SJ, Halsall DJ. Concordance between thyroglobulin antibody assays. Ann Clin Biochem. 2011;48(Pt 4):367-369.
- Rosario PW, Côrtes MCS, Franco Mourão G. Follow-up of patients with thyroid cancer and antithyroglobulin antibodies: a review for clinicians. Endocr Relat Cancer. 2021;28(4):R111-r119.
- van Kinschot CMJ, Peeters RP, van den Berg SAA, Verburg FA, van Noord C, van Ginhoven TM, Visser WE. Thyroglobulin and thyroglobulin antibodies: assay-dependent management consequences in patients with differentiated thyroid carcinoma. Clin Chem Lab Med. 2022;60(5):756-765.
- Ruf J, Carayon P, Lissitzky S. Various expressions of a unique anti-human thyroglobulin antibody repertoire in normal state and autoimmune disease. Eur J Immunol. 1985;15(3):268-272.
- Aras G, Gültekin SS, Küçük NO. The additive clinical value of combined thyroglobulin and antithyroglobulin antibody measurements to define persistent and recurrent disease in patients with differentiated thyroid cancer. Nucl Med Commun. 2008;29(10):880-884.
- Feldt-Rasmussen U, Rasmussen AK. Autoimmunity in differentiated thyroid cancer: significance and related clinical problems. Hormones (Athens). 2010;9(2):109-117.
- Zhao Y, Mu Z, Liang D, Zhang T, Zhang X, Sun D, Sun Y, Liang J, Lin Y. Prognostic value of postoperative anti-thyroglobulin antibody in patients with differentiated thyroid cancer. Front Endocrinol (Lausanne).2024;15:1354426.
- Spencer CA, Takeuchi M, Kazarosyan M, Wang CC, Guttler RB, Singer PA, Fatemi S, LoPresti JS, Nicoloff JT. Serum thyroglobulin autoantibodies: prevalence, influence on serum thyroglobulin measurement, and prognostic significance in patients with differentiated thyroid carcinoma. J Clin Endocrinol Metab. 1998;83(4):1121-1127.
- Görges R, Maniecki M, Jentzen W, Sheu SN, Mann K, Bockisch A, Janssen OE. Development and clinical impact of thyroglobulin antibodies in patients with differentiated thyroid carcinoma during the first 3 years after thyroidectomy. Eur J Endocrinol. 2005;153(1):49-55.
- Kim ES, Lim DJ, Baek KH, Lee JM, Kim MK, Kwon HS, Song KH, Kang MI, Cha BY, Lee KW, Son HY. Thyroglobulin antibody is associated with increased cancer risk in thyroid nodules. Thyroid. 2010;20(8):885-891.
- Ge H, Chen W, Lin Z, Li Y, Chen S. Analysis of the prognostic value of thyroglobulin antibody change trends during follow-up after (131)I treatment in patients with differentiated thyroid carcinoma. Front Oncol.2025;15:1496594.
- Grani G, Calvanese A, Carbotta G, D'Alessandri M, Nesca A, Bianchini M, Del Sordo M, Vitale M, Fumarola A. Thyroid autoimmunity and risk of malignancy in thyroid nodules submitted to fine-needle aspiration cytology. Head Neck. 2015;37(2):260-264.
- Karatzas T, Vasileiadis I, Zapanti E, Charitoudis G, Karakostas E, Boutzios G. Thyroglobulin antibodies as a potential predictive marker of papillary thyroid carcinoma in patients with indeterminate cytology. Am J Surg.2016;212(5):946-952.
- Pacini F, Schlumberger M, Dralle H, Elisei R, Smit JW, Wiersinga W. European consensus for the management of patients with differentiated thyroid carcinoma of the follicular epithelium. Eur J Endocrinol. 2006;154(6):787-803.
- Shin HJ, Lee HS, Kim EK, Moon HJ, Lee JH, Kwak JY. A Study on Serum Antithyroglobulin Antibodies Interference in Thyroglobulin Measurement in Fine-Needle Aspiration for Diagnosing Lymph Node Metastasis in Postoperative Patients. PloS one. 2015;10(6):e0131096.
- Gholve C, Damle A, Kulkarni S, Banerjee S, Rajan M. Evaluation of Different Methods for the Detection of Anti- Thyroglobulin Autoantibody: Prevalence of Anti-Thyroglobulin Autoantibody and Anti-Microsomal Autoantibody in Thyroid Cancer Patients. Indian J Clin Biochem. 2022;37(4):473-479.
- Chiovato L, Latrofa F, Braverman LE, Pacini F, Capezzone M, Masserini L, Grasso L, Pinchera A. Disappearance of humoral thyroid autoimmunity after complete removal of thyroid antigens. Ann Intern Med. 2003;139(5 Pt 1):346-351.
- Kim WG, Yoon JH, Kim WB, Kim TY, Kim EY, Kim JM, Ryu JS, Gong G, Hong SJ, Shong YK. Change of serum antithyroglobulin antibody levels is useful for prediction of clinical recurrence in thyroglobulin-negative patients with differentiated thyroid carcinoma. J Clin Endocrinol Metab. 2008;93(12):4683-4689.
- Scappaticcio L, Trimboli P, Verburg FA, Giovanella L. Significance of "de novo" appearance of thyroglobulin antibodies in patients with differentiated thyroid cancer. Int J Biol Markers. 2020;35(3):41-49.
- Pacini F, Mariotti S, Formica N, Elisei R. Thyroid autoantibodies in thyroid cancer: Incidence and relationship with tumor outcome. Acta Endocrinol. 1988;119:373-380.
- Yamada O, Miyauchi A, Ito Y, Nakayama A, Yabuta T, Masuoka H, Fukushima M, Higashiyama T, Kihara M, Kobayashi K, Miya A. Changes in serum thyroglobulin antibody levels as a dynamic prognostic factor for early-phase recurrence of thyroglobulin antibody-positive papillary thyroid carcinoma after total thyroidectomy. Endocr J.2014;61(10):961-965.
- Gianoukakis AG. Thyroglobulin antibody status and differentiated thyroid cancer: what does it mean for prognosis and surveillance? Curr Opin Oncol. 2015;27(1):26-32.
- Lee ZJO, Eslick GD, Edirimanne S. Investigating Antithyroglobulin Antibody As a Prognostic Marker for Differentiated Thyroid Cancer: A Meta-Analysis and Systematic Review. Thyroid. 2020;30(11):1601-1612.
- Tomer Y, Greenberg D. The thyroglobulin gene as the first thyroid-specific susceptibility gene for autoimmune thyroid disease. Trends Mol Med. 2004;10(7):306-308.
- Vejbjerg P, Knudsen N, Perrild H, Laurberg P, Carlé A, Pedersen IB, Rasmussen LB, Ovesen L, Jørgensen T. Thyroglobulin as a marker of iodine nutrition status in the general population. Eur J Endocrinol. 2009;161(3):475-481.
- Wang Z, Zhang H, Zhang X, Sun J, Han C, Li C, Li Y, Teng X, Fan C, Liu A, Shan Z, Liu C, Weng J, Teng W. Serum thyroglobulin reference intervals in regions with adequate and more than adequate iodine intake. Medicine (Baltimore). 2016;95(48):e5273.
- Bath SC, Pop VJ, Furmidge-Owen VL, Broeren MA, Rayman MP. Thyroglobulin as a Functional Biomarker of Iodine Status in a Cohort Study of Pregnant Women in the United Kingdom. Thyroid. 2017;27(3):426-433.
- Vali M, Rose NR, Caturegli P. Thyroglobulin as autoantigen: structure-function relationships. Rev Endocr Metab Disord. 2000;1(1-2):69-77.
- Citterio CE, Machiavelli GA, Miras MB, Gruneiro-Papendieck L, Lachlan K, Sobrero G, Chiesa A, Walker J, Munoz L, Testa G, Belforte FS, Gonzalez-Sarmiento R, Rivolta CM, Targovnik HM. New insights into thyroglobulin gene: molecular analysis of seven novel mutations associated with goiter and hypothyroidism. Mol Cell Endocrinol.2013;365(2):277-291.
- Persani L, Rurale G, de Filippis T, Galazzi E, Muzza M, Fugazzola L. Genetics and management of congenital hypothyroidism. Best practice & research Clinical endocrinology & metabolism. 2018;32(4):387-396.
- Fernández-Cancio M, Antolín M, Clemente M, Campos-Martorell A, Mogas E, Baz-Redón N, Leno-Colorado J, Comas-Armangué G, García-Arumí E, Soler-Colomer L, González-Llorens N, Camats-Tarruella N, Yeste D. Clinical and molecular study of patients with thyroid dyshormogenesis and variants in the thyroglobulin gene. Front Endocrinol (Lausanne). 2024;15:1367808.
- Chow E, Siddique F, Gama R. Thyrotoxicosis factitia: role of thyroglobulin. Ann Clin Biochem. 2008;45(Pt 4):447-448; author reply 448.
- Jahagirdar VR, Strouhal P, Holder G, Gama R, Singh BM. Thyrotoxicosis factitia masquerading as recurrent Graves' disease: endogenous antibody immunoassay interference, a pitfall for the unwary. Ann Clin Biochem.2008;45(Pt 3):325-327.
- Vorasart P, Sriphrapradang C. Factitious thyrotoxicosis: how to find it. Diagnosis (Berl). 2020;7(2):141-145.
- Bögershausen LR, Giovanella L, Stief T, Luster M, Verburg FA. Long-term predictive value of highly sensitive thyroglobulin measurement. Clin Endocrinol (Oxf). 2023;98(4):622-628.
- Giovanella L, Milan L, Roll W, Weber M, Schenke S, Kreissl M, Vrachimis A, Pabst K, Murat T, Petranović Ovčariček P, Campenni A, Görges R, Ceriani L. Thyroglobulin measurement is the most powerful outcome predictor in differentiated thyroid cancer: a decision tree analysis in a European multicenter series. Clin Chem Lab Med. 2024;62(11):2307-2315.
- Giovanella L, D'Aurizio F, Petranović Ovčariček P, Görges R. Diagnostic, Theranostic and Prognostic Value of Thyroglobulin in Thyroid Cancer. J Clin Med. 2024;13(9).
- Chindris AM, Diehl NN, Crook JE, Fatourechi V, Smallridge RC. Undetectable sensitive serum thyroglobulin (<0.1 ng/ml) in 163 patients with follicular cell-derived thyroid cancer: results of rhTSH stimulation and neck ultrasonography and long-term biochemical and clinical follow-up. J Clin Endocrinol Metab. 2012;97(8):2714-2723.
- Trimboli P, La Torre D, Ceriani L, Condorelli E, Laurenti O, Romanelli F, Ventura C, Signore A, Valabrega S, Giovanella L. High sensitive thyroglobulin assay on thyroxine therapy: can it avoid stimulation test in low and high risk differentiated thyroid carcinoma patients? Horm Metab Res. 2013;45(9):664-668.
- Giovanella L, Castellana M, Trimboli P. Unstimulated high-sensitive thyroglobulin is a powerful prognostic predictor in patients with thyroid cancer. Clin Chem Lab Med. 2019;58(1):130-137.
- Fernández-Velasco P, Díaz-Soto G, Pérez López P, Torres Torres B, de Luis D. Predictive value and dynamic risk stratification of high sensitive basal or stimulated thyroglobulin assay in a long-term thyroid carcinoma cohort. Endocrine. 2023;81(1):116-122.
- Spencer C, Petrovic I, Fatemi S, LoPresti J. Serum thyroglobulin (Tg) monitoring of patients with differentiated thyroid cancer using sensitive (second-generation) immunometric assays can be disrupted by false-negative and false-positive serum thyroglobulin autoantibody misclassifications. J Clin Endocrinol Metab. 2014;99(12):4589-4599.
- Latrofa F, Ricci D, Sisti E, Piaggi P, Nencetti C, Marinò M, Vitti P. Significance of Low Levels of Thyroglobulin Autoantibodies Associated with Undetectable Thyroglobulin After Thyroidectomy for Differentiated Thyroid Carcinoma. Thyroid. 2016;26(6):798-806.
- Jindal A, Khan U. Is Thyroglobulin Level by Liquid Chromatography Tandem-Mass Spectrometry Always Reliable for Follow-Up of DTC After Thyroidectomy: A Report on Two Patients. Thyroid. 2016;26(9):1334-1335.
- Azmat U, Porter K, Senter L, Ringel MD, Nabhan F. Thyroglobulin Liquid Chromatography-Tandem Mass Spectrometry Has a Low Sensitivity for Detecting Structural Disease in Patients with Antithyroglobulin Antibodies. Thyroid. 2017;27(1):74-80.
- Spencer CA, Wang CC. Thyroglobulin measurement. Techniques, clinical benefits, and pitfalls. Endocrinol Metab Clin North Am. 1995;24(4):841-863.
- Ross HA, Netea-Maier RT, Schakenraad E, Bravenboer B, Hermus AR, Sweep FC. Assay bias may invalidate decision limits and affect comparability of serum thyroglobulin assay methods: an approach to reduce interpretation differences. Clin Chim Acta. 2008;394(1-2):104-109.
- Spencer C, Fatemi S, Singer P, Nicoloff J, Lopresti J. Serum Basal thyroglobulin measured by a second-generation assay correlates with the recombinant human thyrotropin-stimulated thyroglobulin response in patients treated for differentiated thyroid cancer. Thyroid. 2010;20(6):587-595.
- Giovanella L, Clark PM, Chiovato L, Duntas L, Elisei R, Feldt-Rasmussen U, Leenhardt L, Luster M, Schalin-Jäntti C, Schott M, Seregni E, Rimmele H, Smit J, Verburg FA. Thyroglobulin measurement using highly sensitive assays in patients with differentiated thyroid cancer: a clinical position paper. Eur J Endocrinol. 2014;171(2):R33-46.
- Iervasi A, Iervasi G, Ferdeghini M, Solimeo C, Bottoni A, Rossi L, Colato C, Zucchelli GC. Clinical relevance of highly sensitive Tg assay in monitoring patients treated for differentiated thyroid cancer. Clin Endocrinol (Oxf).2007;67(3):434-441.
- Bachelot A, Cailleux AF, Klain M, Baudin E, Ricard M, Bellon N, Caillou B, Travagli JP, Schlumberger M. Relationship between tumor burden and serum thyroglobulin level in patients with papillary and follicular thyroid carcinoma. Thyroid. 2002;12(8):707-711.
- Giovanella L, Imperiali M, Ferrari A, Palumbo A, Furlani L, Graziani MS, Castello R. Serum thyroglobulin reference values according to NACB criteria in healthy subjects with normal thyroid ultrasound. Clin Chem Lab Med.2012;50(5):891-893.
- Sobrero G, Munoz L, Bazzara L, Martin S, Silvano L, Iorkansky S, Bergoglio L, Spencer C, Miras M. Thyroglobulin reference values in a pediatric infant population. Thyroid. 2007;17(11):1049-1054.
- Sands NB, Karls S, Rivera J, Tamilia M, Hier MP, Black MJ, Gologan O, Payne RJ. Preoperative serum thyroglobulin as an adjunct to fine-needle aspiration in predicting well-differentiated thyroid cancer. J Otolaryngol Head Neck Surg. 2010;39(6):669-673.
- Trimboli P, Treglia G, Giovanella L. Preoperative measurement of serum thyroglobulin to predict malignancy in thyroid nodules: a systematic review. Horm Metab Res. 2015;47(4):247-252.
- Jo K, Kim MH, Ha J, Lim Y, Lee S, Bae JS, Jung CK, Kang MI, Cha BY, Lim DJ. Prognostic value of preoperative anti-thyroglobulin antibody in differentiated thyroid cancer. Clin Endocrinol (Oxf). 2017;87(3):292-299.
- Jang A, Jin M, Kim CA, Jeon MJ, Lee YM, Sung TY, Kim TY, Kim WB, Shong YK, Kim WG. Serum thyroglobulin testing after thyroid lobectomy in patients with 1-4 cm papillary thyroid carcinoma. Endocrine. 2023;81(2):290-297.
- Evans C, Lotz J, Bhandari M, Hellier RT, Wang XY, Lott R, Lackner KJ, Müller R, Kulasingam V. Multi-center evaluation of the highly sensitive Abbott ARCHITECT and Alinity thyroglobulin chemiluminescent microparticle immunoassay. J Clin Lab Anal. 2022;36(9):e24595.
- Heilig B, Hufner M, Dorken B, Schmidt-Gayk H. Increased heterogeneity of serum thyroglobulin in thyroid cancer patients as determined by monoclonal antibodies. Klin Wochenschr. 1986;64:776-780.
- Schulz R, Bethauser H, Stempka L, Heilig B, Moll A, Hufner M. Evidence for immunological differences between circulating and tissue-derived thyroglobulin in men. Eur J Clin Invest. 1989;19:459-463.
- Magro G, Perissinotto D, Schiappacassi M, Goletz S, Otto A, Müller EC, Bisceglia M, Brown G, Ellis T, Grasso S, Colombatti A, Perris R. Proteomic and postproteomic characterization of keratan sulfate-glycanated isoforms of thyroglobulin and transferrin uniquely elaborated by papillary thyroid carcinomas. Am J Pathol. 2003;163(1):183-196.
- Schlumberger M, Hitzel A, Toubert ME, Corone C, Troalen F, Schlageter MH, Claustrat F, Koscielny S, Taieb D, Toubeau M, Bonichon F, Borson-Chazot F, Leenhardt L, Schvartz C, Dejax C, Brenot-Rossi I, Torlontano M, Tenenbaum F, Bardet S, Bussière F, Girard JJ, Morel O, Schneegans O, Schlienger JL, Prost A, So D, Archambeaud F, Ricard M, Benhamou E. Comparison of seven serum thyroglobulin assays in the follow-up of papillary and follicular thyroid cancer patients. J Clin Endocrinol Metab. 2007;92(7):2487-2495.
- Shaw JB, Harvey SR, Du C, Xu Z, Edgington RM, Olmedillas E, Saphire EO, Wysocki VH. Protein Complex Heterogeneity and Topology Revealed by Electron Capture Charge Reduction and Surface Induced Dissociation. ACS Cent Sci. 2024;10(8):1537-1547.
- Jensen E, Petersen PH, Blaabjerg O, Hegedüs L. Biological variation of thyroid autoantibodies and thyroglobulin. Clin Chem Lab Med. 2007;45(8):1058-1064.
- Spencer CA, LoPresti JS. Measuring thyroglobulin and thyroglobulin autoantibody in patients with differentiated thyroid cancer. Nature clinical practice Endocrinology & metabolism. 2008;4(4):223-233.
- Grebe S. Soluble thyroid tumor markers – old and new challenges and potential solutions. NZ J Med Lab Science 2013;87:76-87.
- Grebe SK. Diagnosis and management of thyroid carcinoma: focus on serum thyroglobulin. Exp Rev Endocrinol Metab. 2009;4:25-43.
- Tomoda C, Miyauchi A. Undetectable serum thyroglobulin levels in patients with medullary thyroid carcinoma after total thyroidectomy without radioiodine ablation. Thyroid. 2012;22(7):680-682.
- Angell TE, Spencer CA, Rubino BD, Nicoloff JT, LoPresti JS. In search of an unstimulated thyroglobulin baseline value in low-risk papillary thyroid carcinoma patients not receiving radioactive iodine ablation. Thyroid.2014;24(7):1127-1133.
- Park S, Jeon MJ, Oh HS, Lee YM, Sung TY, Han M, Han JM, Kim TY, Chung KW, Kim WB, Shong YK, Kim WG. Changes in Serum Thyroglobulin Levels After Lobectomy in Patients with Low-Risk Papillary Thyroid Cancer. Thyroid. 2018;28(8):997-1003.
- Feldt-Rasmussen U, Profilis C, Colinet E, Black E, Bornet H, Bourdoux P, Carayon P, Ericsson UB, Koutras DA, Lamas de Leon L, DeNayer P, Pacini F, Palumbo G, Santos A, Schlumberger M, Seidel C, Van Herle AJ, JJM D. Human thyroglobulin reference material (CRM 457) 1st part: Assessment of homogeneity, stability and immunoreactivity. Ann Biol Clin. 1996;54:337-342.
- Feldt-Rasmussen U, Profilis C, Colinet E, Black E, Bornet H, Bourdoux P, Carayon P, Ericsson UB, Koutras DA, Lamas de Leon L, DeNayer P, Pacini F, Palumbo G, Santos A, Schlumberger M, Seidel C, Van Herle AJ, JJM D. Human thyroglobulin reference material (CRM 457) 2nd part: Physicochemical characterization and certification. Ann Biol Clin. 1996;54:343-348.
- Cubero JM, Rodríguez-Espinosa J, Gelpi C, Estorch M, Corcoy R. Thyroglobulin autoantibody levels below the cut-off for positivity can interfere with thyroglobulin measurement. Thyroid. 2003;13(7):659-661.
- Malandrino P, Tumino D, Russo M, Marescalco S, Fulco RA, Frasca F. Surveillance of patients with differentiated thyroid cancer and indeterminate response: a longitudinal study on basal thyroglobulin trend. J Endocrinol Invest.2019;42(10):1223-1230.
- Cole TG, Johnson D, Eveland BJ, Nahm MH. Cost-effective method for detection of "hook effect" in tumor marker immunometric assays. Clin Chem. 1993;39:695-696.
- Jassam N, Jones CM, Briscoe T, Horner JH. The hook effect: a need for constant vigilance. Ann Clin Biochem.2006;43(Pt 4):314-317.
- Leboeuf R, Langlois MF, Martin M, Ahnadi CE, Fink GD. "Hook effect" in calcitonin immunoradiometric assay in patients with metastatic medullary thyroid carcinoma: case report and review of the literature. J Clin Endocrinol Metab. 2006;91(2):361-364.
- Hillebrand JJ, Siegelaar SE, Heijboer AC. Falsely decreased thyroglobulin levels in a patient with differentiated thyroid carcinoma. Clin Chim Acta. 2020;509:217-219.
- Giovanella L, Ghelfo A. Undetectable serum thyroglobulin due to negative interference of heterophile antibodies in relapsing thyroid carcinoma. Clin Chem. 2007;53(10):1871-1872.
- Ross HA, Menheere PP, Thomas CM, Mudde AH, Kouwenberg M, Wolffenbuttel BH. Interference from heterophilic antibodies in seven current TSH assays. Ann Clin Biochem. 2008;45(Pt 6):616.
- Ding L, Shankara-Narayana N, Wood C, Ward P, Sidhu S, Clifton-Bligh R. Markedly elevated serum thyroglobulin associated with heterophile antibodies: a cautionary tale. Thyroid. 2013;23(6):771-772.
- Netzel BC, Grebe SK, Algeciras-Schimnich A. Usefulness of a thyroglobulin liquid chromatography-tandem mass spectrometry assay for evaluation of suspected heterophile interference. Clin Chem. 2014;60(7):1016-1018.
- Guastapaglia L, Chiamolera MI, Viana Lima Junior J, Ferrer CMF, Godoy Viana L, Veiga Chang C, Andrade Siqueira R, Monteiro Barros Maciel R, Henriques Vieira JG, Biscolla RPM. False diagnosis of recurrent thyroid carcinoma: the importance of testing for heterophile antibodies. Archives of endocrinology and metabolism.2024;68:e230115.
- Cheng F, Chen J, Wu BL, Yang X, Huang YS. A Rare Case of Falsely Elevated High-Sensitivity Cardiac Troponin T due to Antibody Interference and a Literature Review. Clin Lab. 2023;69(8).
- Spencer CA. Recoveries cannot be used to authenticate thyroglobulin (Tg) measurements when sera contain Tg autoantibodies. Clin Chem. 1996;42(5):661-663.
- Weigle WO, High GJ. The behaviour of autologous thyroglobulin in the circulation of rabbits immunized with either heterologous or altered homologous thyroglobulin. J Immunol. 1967;98:1105-1114.
- Feldt-Rasmussen U. Serum thyroglobulin and thyroglobulin autoantibodies in thyroid diseases. Pathogenic and diagnostic aspects. Allergy. 1983;38(6):369-387.
- Sellitti DF, Suzuki K. Intrinsic regulation of thyroid function by thyroglobulin. Thyroid. 2014;24(4):625-638.
- Igawa T, Haraya K, Hattori K. Sweeping antibody as a novel therapeutic antibody modality capable of eliminating soluble antigens from circulation. Immunological reviews. 2016;270(1):132-151.
- Latrofa F, Ricci D, Bottai S, Brozzi F, Chiovato L, Piaggi P, Marinò M, Vitti P. Effect of Thyroglobulin Autoantibodies on the Metabolic Clearance of Serum Thyroglobulin. Thyroid. 2018;28(3):288-294.
- Tozzoli R, Bizzaro N, Tonutti E, Pradella M, Manoni F, Vilalta D, Bassetti D, Piazza A, Rizzotti P. Immunoassay of anti-thyroid autoantibodies: high analytical variability in second generation methods. Clin Chem Lab Med.2002;40(6):568-573.
- Rosário PW, Maia FF, Fagundes TA, Vasconcelos FP, Cardoso LD, Purisch S. Antithyroglobulin antibodies in patients with differentiated thyroid carcinoma: methods of detection, interference with serum thyroglobulin measurement and clinical significance. Arquivos brasileiros de endocrinologia e metabologia. 2004;48(4):487-492.
- Feldt-Rasmussen U, Petersen PH, Date J, Madsen CM. Sequential changes in serum thyroglobulin (Tg) and its autoantibodies (TgAb) following subtotal thyroidectomy of patients with preoperatively detectable TgAb. Clin Endocrinol. 1980;12:29-38.
- Richards DB, Cookson LM, Berges AC, Barton SV, Lane T, Ritter JM, Fontana M, Moon JC, Pinzani M, Gillmore JD, Hawkins PN, Pepys MB. Therapeutic Clearance of Amyloid by Antibodies to Serum Amyloid P Component. N Engl J Med. 2015;373(12):1106-1114.
- Schneider AB, Pervos R. Radioimmunoassay of human thyroglobulin: effect of antithyroglobulin autoantibodies. J Clin Endocrinol Metab. 1978;47:126-137.
- Feldt-Rasmussen U, Rasmussen A K. Serum thyroglobulin (Tg)in presence of thyroglobulin autoantibodies (TgAb). Clinical and methodological relevance of the interaction between Tg and TgAb in vivo and in vitro. J Endocrinol Invest. 1985;8:571-576.
- Benvenga S, Burek CL, Talor M, Rose NR, Trimarchi F. Heterogeneity of the thyroglobulin epitopes associated with circulating thyroid hormone autoantibodies in hashimoto's thyroiditis and non-autoimmune thyroid diseases. J Endocrinol Invest. 2002;25(11):977-982.
- Crane MS, Strachan MW, Toft AD, Beckett GJ. Discordance in thyroglobulin measurements by radioimmunoassay and immunometric assay: a useful means of identifying thyroglobulin assay interference. Ann Clin Biochem.2013;50(Pt 5):421-432.
- Spencer C, Fatemi S. Thyroglobulin antibody (TgAb) methods - Strengths, pitfalls and clinical utility for monitoring TgAb-positive patients with differentiated thyroid cancer. Best practice & research Clinical endocrinology & metabolism. 2013;27(5):701-712.
- Van Herle AJ, Uller RP. Elevated serum thyroglobulin: a marker of metastases in differentiated thyroid carcinomas. J Clin Invest. 1975;56:272-277.
- Spencer CA, Platler BW, Nicoloff JT. The effect of 125-I thyroglobulin tracer heterogeneity on serum Tg RIA measurement. Clin Chim Acta. 1985;153:105-115.
- Spencer CA, Platler B, Guttler RB, Nicoloff JT. Heterogeneity of 125-I labelled thyroglobulin preparations. Clin Chim Acta. 1985;151:121-132.
- Black EG, Hoffenberg R. Should one measure serum thyroglobulin in the presence of anti-thyroglobulin antibodies? Clin Endocrinol. 1983;19:597-601.
- Mariotti S, Barbesino G, Caturegli P, Marino M, Manetti L, Pacini F, Centoni R, Pinchera A. Assay of thyroglobulin in serum with thyroglobulin autoantibodies: an unobtainable goal? J Clin Endocrinol Metab. 1995;80:468-472.
- Gholve C, Kumarasamy J, Damle A, Kulkarni S, Venkatesh M, Banerjee S, Rajan MGR. Comparison of Serum Thyroglobulin Levels in Differentiated Thyroid Cancer Patients Using In-House Developed Radioimmunoassay and Immunoradiometric Procedures. Indian J Clin Biochem. 2019;34(4):465-471.
- L E M Miles CNH. Labelled antibodies and immunological assay systems. Nature. 1968;219:186-189.
- Latrofa F, Ricci D, Grasso L, Vitti P, Masserini L, Basolo F, Ugolini C, Mascia G, Lucacchini A, Pinchera A. Characterization of thyroglobulin epitopes in patients with autoimmune and non-autoimmune thyroid diseases using recombinant human monoclonal thyroglobulin autoantibodies. J Clin Endocrinol Metab. 2008;93(2):591-596.
- Shuford CM, Johnson JS, Thompson JW, Holland PL, Hoofnagle AN, Grant RP. More sensitivity is always better: Measuring sub-clinical levels of serum thyroglobulin on a µLC-MS/MS system. Clin Mass Spectrom. 2020;15:29-35.
- Feldt-Rasmussen U, Verburg FA, Luster M, Cupini C, Chiovato L, Duntas L, Elisei R, Rimmele H, Seregni E, Smit JW, Theimer C, Giovanella L. Thyroglobulin autoantibodies as surrogate biomarkers in the management of patients with differentiated thyroid carcinoma. Curr Med Chem. 2014;21(32):3687-3692.
- Hsieh CJ, Wang PW. Sequential changes of serum antithyroglobulin antibody levels are a good predictor of disease activity in thyroglobulin-negative patients with papillary thyroid carcinoma. Thyroid. 2014;24(3):488-493.
- Matrone A, Latrofa F, Torregrossa L, Piaggi P, Gambale C, Faranda A, Ricci D, Agate L, Molinaro E, Basolo F, Vitti P, Elisei R. Changing Trend of Thyroglobulin Antibodies in Patients With Differentiated Thyroid Cancer Treated With Total Thyroidectomy Without (131)I Ablation. Thyroid. 2018;28(7):871-879.
- Thomas D, Liakos V, Vassiliou E, Hatzimarkou F, Tsatsoulis A, Kaldrimides P. Possible reasons for different pattern disappearance of thyroglobulin and thyroid peroxidase autoantibodies in patients with differentiated thyroid carcinoma following total thyroidectomy and iodine-131 ablation. J Endocrinol Invest. 2007;30(3):173-180.
- Hammarlund E, Thomas A, Amanna IJ, Holden LA, Slayden OD, Park B, Gao L, Slifka MK. Plasma cell survival in the absence of B cell memory. Nat Commun. 2017;8(1):1781.
- Tsushima Y, Miyauchi A, Ito Y, Kudo T, Masuoka H, Yabuta T, Fukushima M, Kihara M, Higashiyama T, Takamura Y, Kobayashi K, Miya A, Kikumori T, Imai T, Kiuchi T. Prognostic significance of changes in serum thyroglobulin antibody levels of pre- and post-total thyroidectomy in thyroglobulin antibody-positive papillary thyroid carcinoma patients. Endocr J. 2013;60(7):871-876.
- Uller RP, Van Herle AJ. Effect of therapy on serum thyroglobulin levels in patients with Graves' disease. J Clin Endocrinol Metab. 1978;46:747-755.
- Feldt-Rasmussen U, Blichert-Toft M, Christiansen C, Date J. Serum thyroglobulin and its autoantibody following subtotal thyroid resection of Graves' disease. Eur J Clin Invest. 1982;12(3):203-208.
- Benvenga S, Bartolone L, Squadrito S, Trimarchi F. Thyroid hormone autoantibodies elicited by diagnostic fine needle biopsy. J Clin Endocrinol Metab. 1997;82(12):4217-4223.
- Polyzos SA, Anastasilakis AD. Alterations in serum thyroid-related constituents after thyroid fine-needle biopsy: a systematic review. Thyroid. 2010;20(3):265-271.
- Al-Hilli Z, Strajina V, McKenzie TJ, Thompson GB, Farley DR, Regina Castro M, Algeciras-Schimnich A, Richards ML. Thyroglobulin Measurement in Fine-Needle Aspiration Improves the Diagnosis of Cervical Lymph Node Metastases in Papillary Thyroid Carcinoma. Ann Surg Oncol. 2017;24(3):739-744.
- Feldt-Rasmussen U, Bech K, Date J, Hyltoft Pedersen P, Johansen K, Nistrup Madsen S. Thyroid stimulating antibodies, thyroglobulin antibodies and serum proteins during treatment of Graves' disease with radioiodine or propylthiouracil. Allergy. 1982;37(3):161-167.
- Feldt-Rasmussen U, Bech K, Date J, Petersen PH, Johansen K. A prospective study of the differential changes in serum thyroglobulin and its autoantibodies during propylthiouracil or radioiodine therapy of patients with Graves' disease. Acta Endocrinol (Copenh). 1982;99(3):379-385.
- Yin N, Sherman SI, Pak Y, Litofsky DR, Gianoukakis AG. The De Novo Detection of Anti-Thyroglobulin Antibodies and Differentiated Thyroid Cancer Recurrence. Thyroid. 2020;30(10):1490-1495.
- Donegan D, McIver B, Algeciras-Schimnich A. Clinical consequences of a change in anti-thyroglobulin antibody assays during the follow-up of patients with differentiated thyroid cancer. Endocr Pract. 2014;20(10):1032-1036.
- Davies L, Welch HG. Epidemiology of head and neck cancer in the United States. Otolaryngol Head Neck Surg.2006;135(3):451-457.
- Pellegriti G, Frasca F, Regalbuto C, Squatrito S, Vigneri R. Worldwide increasing incidence of thyroid cancer: update on epidemiology and risk factors. J Cancer Epidemiol. 2013;2013:965212.
- Lim H, Devesa SS, Sosa JA, Check D, Kitahara CM. Trends in Thyroid Cancer Incidence and Mortality in the United States, 1974-2013. Jama. 2017;317(13):1338-1348.
- Bell R, Weinberger DM, Venkatesh M, Fernandes-Taylor S, Francis DO, Davies L. Thyroid Cancer Incidence During 2020 to 2021 COVID-19 Variant Waves. JAMA Otolaryngol Head Neck Surg. 2024;150(11):969-977.
- Morosán YJ, Parisi C, Urrutia MA, Rosmarin M, Schnitman M, Serrano L, Luciani W, Faingold C, Pitoia F, Brenta G. Dynamic prediction of the risk of recurrence in patients over 60 years of age with differentiated thyroid carcinoma. Archives of endocrinology and metabolism. 2016;60(4):348-354.
- Hay ID, Johnson TR, Kaggal S, Reinalda MS, Iniguez-Ariza NM, Grant CS, Pittock ST, Thompson GB. Papillary Thyroid Carcinoma (PTC) in Children and Adults: Comparison of Initial Presentation and Long-Term Postoperative Outcome in 4432 Patients Consecutively Treated at the Mayo Clinic During Eight Decades (1936-2015). World J Surg. 2018;42(2):329-342.
- Sanabria A, Ferraz C, Ku CHC, Padovani R, Palacios K, Paz JL, Roman A, Smulever A, Vaisman F, Pitoia F. Implementing active surveillance for low-risk thyroid carcinoma into clinical practice: collaborative recommendations for Latin America. Archives of endocrinology and metabolism. 2024;68:e230371.
- Maniam P, Hey SY, Evans-Harding N, Li L, Conn B, Adamson RM, Hay AJ, Lyall M, Nixon IJ. Practice patterns in management of differentiated thyroid cancer since the 2014 British Thyroid Association (BTA) guidelines. Surgeon.2024;22(1):e54-e60.
- Smallridge RC, Diehl N, Bernet V. Practice trends in patients with persistent detectable thyroglobulin and negative diagnostic radioiodine whole body scans: a survey of American Thyroid Association members. Thyroid.2014;24(10):1501-1507.
- Rinaldi S, Plummer M, Biessy C, Tsilidis KK, Østergaard JN, Overvad K, Tjønneland A, Halkjaer J, Boutron-Ruault MC, Clavel-Chapelon F, Dossus L, Kaaks R, Lukanova A, Boeing H, Trichopoulou A, Lagiou P, Trichopoulos D, Palli D, Agnoli C, Tumino R, Vineis P, Panico S, Bueno-de-Mesquita HB, Peeters PH, Weiderpass E, Lund E, Quirós JR, Agudo A, Molina E, Larrañaga N, Navarro C, Ardanaz E, Manjer J, Almquist M, Sandström M, Hennings J, Khaw KT, Schmidt J, Travis RC, Byrnes G, Scalbert A, Romieu I, Gunter M, Riboli E, Franceschi S. Thyroid-stimulating hormone, thyroglobulin, and thyroid hormones and risk of differentiated thyroid carcinoma: the EPIC study. J Natl Cancer Inst. 2014;106(6):dju097.
- Petric R, Besic H, Besic N. Preoperative serum thyroglobulin concentration as a predictive factor of malignancy in small follicular and Hürthle cell neoplasms of the thyroid gland. World J Surg Oncol. 2014;12:282.
- Tian D, Li X, Jia Z. Analysis of Risk Factors and Risk Prediction for Cervical Lymph Node Metastasis in Thyroid Papillary Carcinoma. Cancer Manag Res. 2024;16:1571-1585.
- Rosignolo F, Maggisano V, Sponziello M, Celano M, Di Gioia CR, D'Agostino M, Giacomelli L, Verrienti A, Dima M, Pecce V, Durante C. Reduced expression of THRβ in papillary thyroid carcinomas: relationship with BRAF mutation, aggressiveness and miR expression. J Endocrinol Invest. 2015;38(12):1283-1289.
- Giovanella L, Ceriani L, Ghelfo A, Maffioli M, Keller F. Preoperative undetectable serum thyroglobulin in differentiated thyroid carcinoma: incidence, causes and management strategy. Clin Endocrinol (Oxf).2007;67(4):547-551.
- Durante C, Puxeddu E, Ferretti E, Morisi R, Moretti S, Bruno R, Barbi F, Avenia N, Scipioni A, Verrienti A, Tosi E, Cavaliere A, Gulino A, Filetti S, Russo D. BRAF mutations in papillary thyroid carcinomas inhibit genes involved in iodine metabolism. J Clin Endocrinol Metab. 2007;92(7):2840-2843.
- Tallini G, de Biase D, Durante C, Acquaviva G, Bisceglia M, Bruno R, Bacchi Reggiani ML, Casadei GP, Costante G, Cremonini N, Lamartina L, Meringolo D, Nardi F, Pession A, Rhoden KJ, Ronga G, Torlontano M, Verrienti A, Visani M, Filetti S. BRAF V600E and risk stratification of thyroid microcarcinoma: a multicenter pathological and clinical study. Mod Pathol. 2015;28(10):1343-1359.
- Haugen BR, Ladenson PW, Cooper DS, Pacini F, Reiners C, Luster M, Schlumberger M, Sherman SI, Samuels M, Graham K, Braverman LE, Skarulis MC, Davies TF, DeGroot L, Mazzaferri EL, Daniels GH, Ross DC, Becker DV, Mazon HR, Cavalieri RR, Spencer CA, McEllin K, Weintraub BD, EC. R. A comparison of Recombinant Human Thyrotropin and Thyroid Hormone Withdrawal for the Detection of Thyroid Remnant or Cancer. J Clin Endocrinol Metab. 1999;84:3877-3885.
- Giovanella L, Ceriani L, Suriano S, Ghelfo A, Maffioli M. Thyroglobulin measurement before rhTSH-aided 131I ablation in detecting metastases from differentiated thyroid carcinoma. Clin Endocrinol (Oxf). 2008;69(4):659-663.
- Zhu Y, Yang X, Liu Z, Zhang Q, Li Z, Hou X, Zhu H. Predictive value of thyroglobulin after radioiodine therapy for excellent response to treatment in postoperative thyroid cancer. Nucl Med Commun. 2024.
- Feldt-Rasmussen U, Petersen PH, Nielsen H, Date J. Thyroglobulin of varying molecular sizes with different disappearence rates in plasma following subtotal thyroidectomy. Clin Endocrinol (Oxf). 1978;9:205-?????
- Durante C, Montesano T, Attard M, Torlontano M, Monzani F, Costante G, Meringolo D, Ferdeghini M, Tumino S, Lamartina L, Paciaroni A, Massa M, Giacomelli L, Ronga G, Filetti S. Long-term surveillance of papillary thyroid cancer patients who do not undergo postoperative radioiodine remnant ablation: is there a role for serum thyroglobulin measurement? J Clin Endocrinol Metab. 2012;97(8):2748-2753.
- Padovani RP, Robenshtok E, Brokhin M, Tuttle RM. Even without additional therapy, serum thyroglobulin concentrations often decline for years after total thyroidectomy and radioactive remnant ablation in patients with differentiated thyroid cancer. Thyroid. 2012;22(8):778-783.
- Tuttle RM, Leboeuf R. Follow up approaches in thyroid cancer: a risk adapted paradigm. Endocrinol Metab Clin North Am. 2008;37(2):419-435, ix-x.
- Miyauchi A, Kudo T, Miya A, Kobayashi K, Ito Y, Takamura Y, Higashiyama T, Fukushima M, Kihara M, Inoue H, Tomoda C, Yabuta T, Masuoka H. Prognostic impact of serum thyroglobulin doubling-time under thyrotropin suppression in patients with papillary thyroid carcinoma who underwent total thyroidectomy. Thyroid.2011;21(7):707-716.
- Couto JS, Almeida MFO, Trindade VCG, Marone MMS, Scalissi NM, Cury AN, Ferraz C, Padovani RP. A cutoff thyroglobulin value suggestive of distant metastases in differentiated thyroid cancer patients. Braz J Med Biol Res.2020;53(11):e9781.
- Pacini F, Sabra MM, Tuttle RM. Clinical relevance of thyroglobulin doubling time in the management of patients with differentiated thyroid cancer. Thyroid. 2011;21(7):691-692.
- Miyauchi A, Kudo T, Kihara M, Higashiyama T, Ito Y, Kobayashi K, Miya A. Relationship of biochemically persistent disease and thyroglobulin-doubling time to age at surgery in patients with papillary thyroid carcinoma. Endocr J.2013;60(4):415-421.
- Giovanella L, Trimboli P, Verburg FA, Treglia G, Piccardo A, Foppiani L, Ceriani L. Thyroglobulin levels and thyroglobulin doubling time independently predict a positive 18F-FDG PET/CT scan in patients with biochemical recurrence of differentiated thyroid carcinoma. Eur J Nucl Med Mol Imaging. 2013;40(6):874-880.
- Giovanella L, Garo ML, Albano D, Görges R, Ceriani L. The role of thyroglobulin doubling time in differentiated thyroid cancer: a meta-analysis. Endocr Connect. 2022;11(4).
- Ito Y, Miyauchi A. Prognostic factors of papillary and follicular carcinomas based on pre-, intra-, and post-operative findings. Eur Thyroid J. 2024;13(5).
- Schlumberger M CP, Fragu P, Lumbroso J, Parmentier C and Tubiana M,. Circulating thyrotropin and thyroid hormones in patients with metastases of differentiated thyroid carcinoma: relationship to serum thyrotropin levels. J Clin Endocrinol Metab. 1980;51:513-519.
- Robbins RJ, Srivastava S, Shaha A, Ghossein R, Larson SM, Fleisher M, Tuttle RM. Factors influencing the basal and recombinant human thyrotropin-stimulated serum thyroglobulin in patients with metastatic thyroid carcinoma. J Clin Endocrinol Metab. 2004;89(12):6010-6016.
- Cooper DS, Doherty GM, Haugen BR, Kloos RT, Lee SL, Mandel SJ, Mazzaferri EL, McIver B, Sherman SI, Tuttle RM. Management guidelines for patients with thyroid nodules and differentiated thyroid cancer. Thyroid.2006;16(2):109-142.
- Pacini F, Castagna MG. Diagnostic and therapeutic use of recombinant human TSH (rhTSH) in differentiated thyroid cancer. Best practice & research Clinical endocrinology & metabolism. 2008;22(6):1009-1021.
- Mazzaferri EL, Robbins RJ, Spencer CA, Braverman LE, Pacini F, Wartofsky L, Haugen BR, Sherman SI, Cooper DS, Braunstein GD, Lee S, Davies TF, Arafah BM, Ladenson PW, Pinchera A. A consensus report of the role of serum thyroglobulin as a monitoring method for low-risk patients with papillary thyroid carcinoma. J Clin Endocrinol Metab. 2003;88(4):1433-1441.
- Braverman L, Kloos RT, Law B, Jr., Kipnes M, Dionne M, Magner J. Evaluation of various doses of recombinant human thyrotropin in patients with multinodular goiters. Endocr Pract. 2008;14(7):832-839.
- Over R, Nsouli-Maktabi H, Burman KD, Jonklaas J. Age modifies the response to recombinant human thyrotropin. Thyroid. 2010;20(12):1377-1384.
- Smallridge RC, Meek SE, Morgan MA, Gates GS, Fox TP, Grebe S, Fatourechi V. Monitoring thyroglobulin in a sensitive immunoassay has comparable sensitivity to recombinant human tsh-stimulated thyroglobulin in follow-up of thyroid cancer patients. J Clin Endocrinol Metab. 2007;92(1):82-87.
- Mazzaferri EL. Will highly sensitive thyroglobulin assays change the management of thyroid cancer? Clin Endocrinol (Oxf). 2007;67(3):321-323.
- Chindris AM, Casler JD, Bernet VJ, Rivera M, Thomas C, Kachergus JM, Necela BM, Hay ID, Westphal SA, Grant CS, Thompson GB, Schlinkert RT, Thompson EA, Smallridge RC. Clinical and molecular features of Hürthle cell carcinoma of the thyroid. J Clin Endocrinol Metab. 2015;100(1):55-62.
- Benmoussa JA, Chen K, Najjar S, Applewhite M, Warshaw J. Lateral neck Cystic Mass: The Role of Thyroglobulin Measurement in Fine Needle Aspiration. Endocr Pract. 2018;24(8):767.
- Rotman-Pikielny P, Reynolds JC, Barker WC, Yen PM, Skarulis MC, Sarlis NJ. Recombinant human thyrotropin for the diagnosis and treatment of a highly functional metastatic struma ovarii. J Clin Endocrinol Metab.2000;85(1):237-244.
- Russo M, Marturano I, Masucci R, Caruso M, Fornito MC, Tumino D, Tavarelli M, Squatrito S, Pellegriti G. Metastatic malignant struma ovarii with coexistence of Hashimoto's thyroiditis. Endocrinol Diabetes Metab Case Rep. 2016;2016:160030.
- Trimboli P, Guidobaldi L, Bongiovanni M, Crescenzi A, Alevizaki M, Giovanella L. Use of fine-needle aspirate calcitonin to detect medullary thyroid carcinoma: A systematic review. Diagn Cytopathol. 2016;44(1):45-51.
- Randolph GW, Duh QY, Heller KS, LiVolsi VA, Mandel SJ, Steward DL, Tufano RP, Tuttle RM. The prognostic significance of nodal metastases from papillary thyroid carcinoma can be stratified based on the size and number of metastatic lymph nodes, as well as the presence of extranodal extension. Thyroid. 2012;22(11):1144-1152.
- Lin SY, Li MY, Zhou CP, Ao W, Huang WY, Wang SS, Yu JF, Tang ZH, Abdelhamid Ahmed AH, Wang TY, Wang ZH, Hua S, Randolph GW, Zhao WX, Wang B. Accurate preoperative prediction of nodal metastasis in papillary thyroid microcarcinoma: Towards optimal management of patients. Head Neck. 2024;46(5):1009-1019.
- Uruno T, Miyauchi A, Shimizu K, Tomoda C, Takamura Y, Ito Y, Miya A, Kobayashi K, Matsuzuka F, Amino N, Kuma K. Usefulness of thyroglobulin measurement in fine-needle aspiration biopsy specimens for diagnosing cervical lymph node metastasis in patients with papillary thyroid cancer. World J Surg. 2005;29(4):483-485.
- Boi F, Baghino G, Atzeni F, Lai ML, Faa G, Mariotti S. The diagnostic value for differentiated thyroid carcinoma metastases of thyroglobulin (Tg) measurement in washout fluid from fine-needle aspiration biopsy of neck lymph nodes is maintained in the presence of circulating anti-Tg antibodies. J Clin Endocrinol Metab. 2006;91(4):1364-1369.
- Snozek CL, Chambers EP, Reading CC, Sebo TJ, Sistrunk JW, Singh RJ, Grebe SK. Serum thyroglobulin, high-resolution ultrasound, and lymph node thyroglobulin in diagnosis of differentiated thyroid carcinoma nodal metastases. J Clin Endocrinol Metab. 2007;92(11):4278-4281.
- Torres MR, Nóbrega Neto SH, Rosas RJ, Martins AL, Ramos AL, da Cruz TR. Thyroglobulin in the washout fluid of lymph-node biopsy: what is its role in the follow-up of differentiated thyroid carcinoma? Thyroid. 2014;24(1):7-18.
- Tralongo P, Bruno C, Policardo F, Vegni F, Feraco A, Carlino A, Ferraro G, Milardi D, Navarra E, Pontecorvi A, Lombardi CP, Raffaelli M, Larocca LM, Pantanowitz L, Rossi ED. Diagnostic role of FNA cytology in the evaluation of cervical lymph nodes in thyroid cancers: Combined evaluation of thyroglobulin in eluate from FNA cytology. Cancer Cytopathol. 2023;131(11):693-700.
- Grani G, Sponziello M, Filetti S, Durante C. Thyroid nodules: diagnosis and management. Nat Rev Endocrinol.2024;20(12):715-728.
- Sakamoto K, Ozawa H, Sato Y, Nakaishi M, Sakanushi A, Matsunobu T, Okubo K, Shinden S. Cutoff value of thyroglobulin in needle aspirates for screening neck masses of thyroid carcinoma. Endocr Relat Cancer.2024;31(12).
- García-Molina F, Arense-Gonzalo JJ, Aguera-Sanchez A, Peña-Ros E, Ruiz-Marín M, Matínez-Perez M, Chaves-Benito A, Martínez-Diaz F. [Diagnosis of lymph node metastases from papillary thyroid carcinoma by measuring thyroglobulin in the puncture needle. Calculation of optimal cut-off point in our series]. Rev Esp Patol.2024;57(4):258-264.
- Chen J, Lin Z, Xu B, Lu T, Zhang X. The efficacy and assessment value of the level of thyroglobulin wash-out after fine-needle aspiration cytodiagnosis in the evaluation of lymph node metastasis in papillary thyroid carcinoma. World J Surg Oncol. 2024;22(1):149.
- Jeon MJ, Kim WG, Jang EK, Choi YM, Lee YM, Sung TY, Yoon JH, Chung KW, Hong SJ, Baek JH, Lee JH, Kim TY, Shong YK, Kim WB. Thyroglobulin level in fine-needle aspirates for preoperative diagnosis of cervical lymph node metastasis in patients with papillary thyroid carcinoma: two different cutoff values according to serum thyroglobulin level. Thyroid. 2015;25(4):410-416.
- Boi F, Maurelli I, Pinna G, Atzeni F, Piga M, Lai ML, Mariotti S. Calcitonin measurement in wash-out fluid from fine needle aspiration of neck masses in patients with primary and metastatic medullary thyroid carcinoma. J Clin Endocrinol Metab. 2007;92(6):2115-2118.
- Abraham D, Gault PM, Hunt J, Bentz J. Calcitonin estimation in neck lymph node fine-needle aspirate fluid prevents misinterpretation of cytology in patients with metastatic medullary thyroid cancer. Thyroid.2009;19(9):1015-1016.
- Ringel DM, Balducci-Silano PL, Anderson JS, Spencer CA, Silverman J, Sparling YH, Francis GL, Burman KD, Wartofsky L, Ladenson PW, Levine MA, Tuttle RM. Quantitative reverse transcriptase polymerase chain reaction of circulating thyroglobulin messenger RNAfor monitoring patients with thyroid carcinoma. J Clin Endocrinol Metab.1999;84:4037-4042.
- Barzon L, Boscaro M, Pacenti M, Taccaliti A, Palù G. Evaluation of circulating thyroid-specific transcripts as markers of thyroid cancer relapse. Int J Cancer. 2004;110(6):914-920.
- Gupta M, Chia SY. Circulating thyroid cancer markers. Current opinion in endocrinology, diabetes, and obesity.2007;14(5):383-388.
- Grammatopoulos D, Elliott Y, Smith SC, Brown I, Grieve RJ, Hillhouse EW, Levine MA, Ringel MD. Measurement of thyroglobulin mRNA in peripheral blood as an adjunctive test for monitoring thyroid cancer. Mol Pathol.2003;56(3):162-166.
- Ausavarat S, Sriprapaporn J, Satayaban B, Thongnoppakhun W, Laipiriyakun A, Amornkitticharoen B, Chanachai R, Pattanachak C. Circulating thyrotropin receptor messenger ribonucleic acid is not an effective marker in the follow-up of differentiated thyroid carcinoma. Thyroid Res. 2015;8:11.
- Biscolla RP, Cerutti JM, Maciel RM. Detection of recurrent thyroid cancer by sensitive nested reverse transcription-polymerase chain reaction of thyroglobulin and sodium/iodide symporter messenger ribonucleic acid transcripts in peripheral blood. J Clin Endocrinol Metab. 2000;85(10):3623-3627.
- Ringel MD. Molecular detection of thyroid cancer: differentiating "signal" and "noise" in clinical assays. J Clin Endocrinol Metab. 2004;89(1):29-32.
- Kaufmann S, Schmutzler C, Schomburg L, Körber C, Luster M, Rendl J, Reiners C, Köhrle J. Real time RT-PCR analysis of thyroglobulin mRNA in peripheral blood in patients with congenital athyreosis and with differentiated thyroid carcinoma after stimulation with recombinant human thyrotropin. Endocr Regul. 2004;38(2):41-49.
- Chelly J, Concordet JP, Kaplan JC, Kahn A. Illegitimate transcription: transcription of any gene in any cell type. Proc Natl Acad Sci U S A. 1989;86(8):2617-2621.
- Ghossein RA, Bhattacharya S. Molecular detection and characterization of circulating tumor cells and micrometastases in prostatic, urothelial, and renal cell carcinomas. Semin Surg Oncol. 2001;20(4):304-311.
- Savagner F, Rodien P, Reynier P, Rohmer V, Bigorgne JC, Malthiery Y. Analysis of Tg transcripts by real-time RT-PCR in the blood of thyroid cancer patients. J Clin Endocrinol Metab. 2002;87(2):635-639.
- Elisei R, Vivaldi A, Agate L, Molinaro E, Nencetti C, Grasso L, Pinchera A, Pacini F. Low specificity of blood thyroglobulin messenger ribonucleic acid assay prevents its use in the follow-up of differentiated thyroid cancer patients. J Clin Endocrinol Metab. 2004;89(1):33-39.
- Endo T, Kobayashi T. Thyroid-stimulating hormone receptor in brown adipose tissue is involved in the regulation of thermogenesis. Am J Physiol Endocrinol Metab. 2008;295(2):E514-518.
- Cianfarani F, Baldini E, Cavalli A, Marchioni E, Lembo L, Teson M, Persechino S, Zambruno G, Ulisse S, Odorisio T, D'Armiento M. TSH receptor and thyroid-specific gene expression in human skin. J Invest Dermatol.2010;130(1):93-101.
- Campennì A, Aguennouz M, Siracusa M, Alibrandi A, Polito F, Oteri R, Baldari S, Ruggeri RM, Giovanella L. Thyroid Cancer Persistence in Patients with Unreliable Thyroglobulin Measurement: Circulating microRNA as Candidate Alternative Biomarkers. Cancers (Basel). 2022;14(22).
- Fagin JA, Nikiforov YE. Progress in Thyroid Cancer Genomics: A 40-Year Journey. Thyroid. 2023;33(11):1271-1286.