ABSTRACT
Neuroendocrine (NE) cells are rare epithelial cells that, in addition to having an endocrine function, express markers and peptides otherwise associated with neurons and the central nervous system. NE cells can be found as either single cells or small clusters of cells dispersed throughout the parenchymal surface epithelium of different tissues, including the lung, the intestine, and the pancreas. The observation that NE cells, which are dispersed throughout the body in different tissue sites, are often innervated and secrete bioactive compounds that can act both locally and systemically, led to the idea of the diffuse neuroendocrine system, a diffuse hormonal system composed of NE cells. NE cells perform important endocrine functions. Furthermore, NE cells are implicated in several human diseases. In particular, a group of rare tumors that presumably arise from NE cells, neuroendocrine neoplasms (NENs), have sparked a great deal of interest in NE cell biology. NENs can arise in almost all tissues but they show the highest incidence in the lung and the gastroenteropancreatic (GEP) system. In this chapter, we will outline what is currently known about NE cell differentiation and function, focusing specifically on NE cells of the lung, pulmonary neuroendocrine cells (PNECs), and the most prominent NE cells of the GEP system: enteroendocrine cells (EECs) of the small intestine and stomach and pancreatic endocrine cells. We will also discuss the potential role of these specific NE cells in the context of tissue injury. Finally, we will provide a brief overview of NEN biology with regards to NENs arising in the lung and GEP system.
INTRODUCTION
Neuroendocrine (NE) cells are epithelial cells that, in addition to having an endocrine function, express markers and peptides otherwise associated with neurons and the central nervous system (1,2). NE cells can be defined by the presence of dense secretory granules and the expression of general NE markers including chromogranin A and synaptophysin. The first identified NE cells were the enterochromaffin (EC) cells of the small intestine, whose distinctive shape and histological properties piqued the interest of scientists during the late nineteenth and early twentieth centuries. In particular, dense secretory granules within NE cells hint to their endocrine function. These secretory granules also make NE cells reactive to chromium and silver, which makes them easy to identify using histological staining methods. Throughout their history, NE cells in the intestine have been referred to in the literature with a variety of names recalling their distinct reactions to histological stains: clear cells (did not pick up conventional stains), chromaffin cells (reacted to chromium salts), argentaffin cells (affinity for silver stains), and Kulchitsky cells (in honor of one of the scientists who studied them) (3,4).
Following the description of NE cells in the intestine, histological studies revealed the presence of NE cells not only throughout the intestinal mucosa, but also in other epithelial tissues (1–8). NE cells can be found as either single cells or small, often innervated clusters of cells dispersed throughout the parenchymal surface epithelium of different tissues, including the small intestine, the lung, and the urogenital tract. As is the case for the cells of the pancreatic islets of Langerhans, the C cells of the thyroid, and adrenal medullary cells, NE cells can also form distinct clusters of cells within endocrine glands.
In 1938, drawing on his histological studies of NE cells in the pancreas and intestine, Friederich Feyrter proposed that NE cells comprise a diffuse neuroendocrine system. Nearly 30 years later, Anthony Pearse refined this idea of the diffuse neuroendocrine system by showing that NE cells, much like neurons, are able to metabolize amines and produce polypeptide hormones. Thus, the concept of a diffuse neuroendocrine system that functions as a diffuse hormonal system and is composed of cells dispersed throughout the body that, through the secretion of bioactive compounds, communicate in a coordinated fashion with their surroundings and with the nervous system solidified (8,9). Famously, Pearse also suggested that NE cells are all derived from the neural crest. This hypothesis, however, was later disproved by several elegant lineage tracing experiments. With the exception of the cells of the adrenal medulla, the extra-adrenal paraganglia, and C cells of thyroid, which are indeed derived from the neural crest, different types of NE cells are derived from the epithelial progenitors of their respective tissue sites (3).
While the specific function of pancreatic islet cells, the cells of the adrenal medulla, and C cells of the thyroid, for example, have been well-established both in terms of their contribution to specific organ function and the maintenance of homeostasis; the specific functions of other NE cells are less well defined. Furthermore, the list of polypeptide hormones and neuropeptides secreted by NE cells is continuously being updated and further refined. Studies that elucidate the developmental differentiation trajectories of NE cells from different tissues have expanded the list of common NE marker genes so that it no longer includes only hormones and neuropeptides but also lineage specific transcription factors. A summary of common NE markers is provided in Table 1.
Table 1. Common NE Markers
|
NE Marker
|
Function
|
Associated NE cell types
|
ASCL1
|
Transcription factor
|
PNECs, some gastric EECs
|
NEUROD1
|
Transcription factor
|
GEP EECs
|
INSM1
|
Transcription factor
|
All NE cells
|
Chromogranin A (CHGA)*
|
Secretory protein
|
All NE cells
|
Synaptophysin (SYP)*
|
Synaptic vesicle glycoprotein
|
All NE cells
|
NCAM1 (a.k.a. CD56)*
|
Cell adhesion molecule
|
All NE cells
|
UCHL1 (a.k.a. PGP9.5)*
|
Deubiquitinating enzyme
|
All NE cells
|
Neuron Specific Enolase (NSE)*
|
Metabolic enzyme
|
All NE cells
|
* Indicates markers used in clinical diagnosis
Much of the interest in NE cell biology has been initiated by observations that have been made regarding their behavior in disease. In particular, a group of rare tumors that presumably arise from NE cells, neuroendocrine neoplasms (NENs), have sparked a great deal of interest in NE cell biology inasmuch as this might relate to the genesis and peculiar clinical behavior of some of these tumors. NENs have been observed in almost all tissues, and consist of well-differentiated neuroendocrine tumors (NETs), tumors that proliferate and progress slowly, and neuroendocrine carcinomas (NECs), poorly differentiated tumors that have a poor prognosis (10). While NENs were initially classified according to the embryological origin of their tissue site of incidence (i.e., foregut, midgut, or hindgut), they are now referred to according to their specific tissue site of origin and, in the case of tumors that elicit hormonal syndromes, according to the primary hormone they secrete.
NENs show the highest incidence in the lung and the gastroenteropancreatic (GEP) system (Figure 1). For this reason, as outlined above, while there are many different kinds of NE cells arising in many different tissue sites, for the purposes of this chapter, we will focus on the NE cells of the lung, pulmonary neuroendocrine cells (PNECs), and the most prominent NE cells of the GEP system: enteroendocrine cells (EECs) of the small intestine and stomach, and pancreatic islet cells or pancreatic endocrine cells (pECs). These cells are key components of the body’s diffuse hormonal system (Table 2).
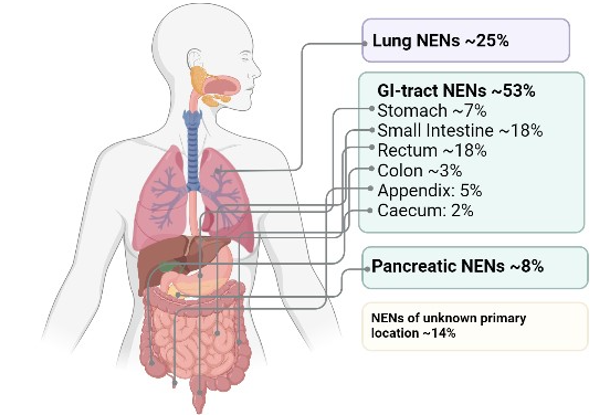
Figure 1. Occurrence of the most common types of neuroendocrine neoplasms. The occurrence of the main types of neuroendocrine tumors presented as the percentage of all NENs (315,441). GI-NENs represent the largest subgroup of NENs, followed by lung and pancreatic NENs. Subtypes not listed in this figure include NENs from the thyroid, kidney, adrenal gland, breast, prostate and skin.
Table 2. Types of NE cells and Their Tissue Site
|
NE cell type
|
Tissue site
|
Predominant hormone
|
PNEC
|
lung
|
numerous (see text)
|
Alpha cells
|
Pancreas
|
Glucagon
|
Beta cells
|
Pancreas
|
Insulin
|
Gamma/ PP cells
|
Pancreas
|
PPY
|
Delta cells
|
Pancreas
|
Somatostatin
|
Epsilon cells
|
Pancreas (during development)
|
Ghrelin
|
G cells
|
Stomach, duodenum, pancreas
|
Gastrin
|
D cells
|
Stomach, small intestine
|
Somatostatin
|
Enterochromaffin cells (EC) cells
|
Stomach, small intestine, colon
|
Serotonin (5-HT)
|
EC-like (ECL) cells
|
Stomach
|
Histamine
|
X and X/A cells
|
Stomach (mainly), small intestine
|
Ghrelin
|
L-I-N lineage cells
|
Small intestine (distal), Colon (L cells)
|
GLP-1, GLP-2, PYY, serotonin (L-cells), CCK, serotonin (I cells), NTS (N cells)
|
K cells
|
Small intestine (proximal)
|
GIP, serotonin
|
PULMONARY NEUROENDOCRINE CELLS (PNECs)
Pulmonary neuroendocrine cells (PNECs), the neuroendocrine cells of the lung, are prominent constituents of the diffuse neuroendocrine system. Although PNECs account for only 0.5% of the lung epithelium, their distinct morphological and histological staining properties, which are shared with the majority of NE cells, led to their prominence in early histological studies of the lung (11). First described in 1949 as “helle zellen” (‘bright cells’ in German), it was later appreciated that PNECs contain secretory granules and that they produce and secrete bioactive compounds, including serotonin (12–14). PNECs were thus added to the growing list of NE cells that make up the diffuse neuroendocrine system.
PNECs are found both as single cells within the lung parenchyma and as small clusters, called neuroendocrine bodies (NEBs) (Figure 2A) (15). In mammalian lung tissue, NEBs are distinctly located next to airway bifurcation points of the branching airways. Solitary PNECs, on the other hand, show a more divergent pattern of localization between species. Whereas in mice, solitary PNECs are mostly found in the trachea, in human lung tissue, they can be found throughout the airways. A distinct feature of PNECs is their direct innervation (16).
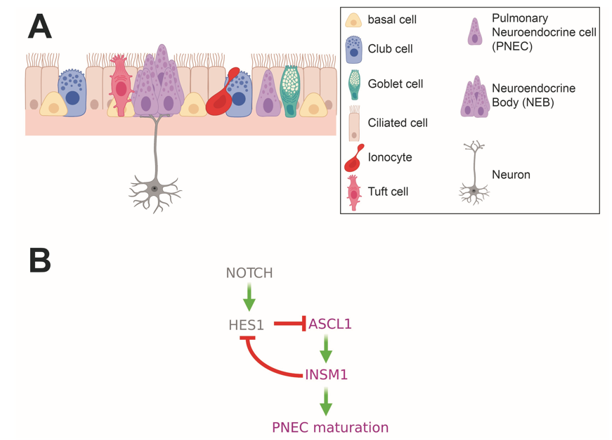
Figure 2. Pulmonary Neuroendocrine Cells (PNECs) in the upper airways. (A) Schematic depicting epithelial cell types found in the upper airways. Solitary PNECs and innervated Neuroendocrine Bodies (NEBs) are shown. (B) Diagram of signaling and transcription factor interactions that regulate PNEC differentiation.
Although the precise function of PNECs is not well-defined, their preservation across evolution -- similar cell types are found in fish gills and in all air-breathing vertebrates -- suggest an important physiological function (17–19). Furthermore, the position at airway bifurcation points of PNECs, their contact with the airway lumen, and innervation suggest a role in airway sensing. The bioactive compounds, hormones, and neuropeptides that PNECs secrete are known to affect oxygen sensing, pulmonary blood flow and bronchial tonus, and lung immune responses. The bioactive compounds secreted by PNECs include serotonin, calcitonin, calcitonin gene-related peptide (CGRP), gastrin-releasing peptide (GRP), chromogranin A, gamma aminobutyric acid (GABA), and synaptophysin, clearly suggesting an endocrine function for these cells (20–23). A summary of the hormones and neuropeptides expressed by PNECs and other NE cells discussed in this text is included in Table 3.
Table 3. NE Cell Expressed Hormones
|
Hormone
|
Associated
NE cell types
|
Reported function in
described NE cell types
|
Calcitonin
|
PNEC
|
pulmonary blood flow and bronchial tonus
|
Calcitonin gene-related peptide (CGRP)
|
PNEC
|
vasoregulation, bronchoprotection, immune cell recruitment
|
Gastrin releasing peptide (GRP)
|
PNEC
|
Regulates mucus and cytokine production upon inflammation or inflammation-associated processes
|
Gamma aminobutyric acid (GABA)
|
PNEC and pECs
|
Regulates mucus and cytokine production upon inflammation or inflammation-associated processes
|
Somatostatin (SST)
|
PNECs and GEP NE cells
(D cells)
|
Inhibits secretion of insulin, glucagon, PYY, serotonin, and gastrin
|
Vasoactive intestinal polypeptide (VIP)
|
PNECs and GEP EECs
|
Acts as a neurotransmitter, an immune regulator, a vasodilator, and a secretagogue
|
Histamine
|
PNECs and Gastric EECs
(ECL cells)
|
Stimulates gastric acid secretion
|
Ghrelin (GHRL)
|
PNECs, Gastric EECs (X/A cell), and pECs (Epsilon cells)
|
Stimulates appetite, promotes gluconeogenesis and increased gastric acid secretion
|
Cholecystokinin (CCK)
|
PNECs and GI EECs
(I cells)
|
Stimulates gallbladder contraction, pancreatic enzyme secretion, gut motility, satiety, and inhibits acid secretion
|
Serotonin (5-HT)
|
PNECs and GI EECs
(EC cells)
|
Regulates vasodilation and smooth muscle contraction
|
Secretin (SCT)
|
GI EECs
|
Stimulates the release of bicarbonate and water to neutralize gastric acid
|
Gastric inhibitory peptide (GIP)*
|
GI EECs (K cells)
|
Inhibits insulin secretion and to a lesser extent gastric acid secretion
|
Substance P (neuropeptide)
|
GI EECs (EC cells)
|
Regulates intestinal motility and mucosal permeability
|
Glucagon-like peptide 1 (GLP-1)
|
Intestinal EECs (L cells)
|
Stimulates insulin secretion and inhibits glucagon secretion
|
Neurotensin (NTS)
|
Intestinal EECs (N cells)
|
Inhibits gastric acid secretion
|
Glucagon (GCG)
|
pECs (alpha cells)
|
Increases blood glucose levels by stimulating glucose production and inhibiting glycogen storage by the liver
|
Insulin (INS)
|
pECs (beta cells)
|
Stimulates uptake of blood glucose by other tissues
|
Gastrin
|
pECs (G cells)
|
Stimulates gastric acid secretion
|
Pancreatic polypeptide (PPY)
|
pECs
(PP/gamma cells)
|
Inhibits glucagon and somatostatin
|
* Gastric inhibitory peptide (GIP) is also known as glucose-dependent insulinotropic polypeptide
PNECs are the first differentiated cell type to appear in the developing lung (15). The timing of PNEC differentiation in human lungs has not been comprehensively delineated but several studies have reported the appearance of PNECs in human fetal airways at 8 - 9 weeks of gestation (24). In mice, the fetal epithelial progenitor cells that give rise to mature PNECs appear for the first time at around embryonic day (E) 12.5 (25). These cells are defined by expression of the basic helix loop helix (bHLH) lineage transcription factor, ASCL1, which is required for their specification. Mice that carry null alleles of Ascl1 do not have PNECs (26,27).
PNEC lineage specification by expression of Ascl1 is followed by two key events that appear to happen in parallel: the formation of NEBs and the maturation of early Ascl1 expressing cells to fully differentiated PNECs. Using a lineage trace of Ascl1-positive cells in mouse embryonic lung, Kuo and Krasnow observed the first signs of PNEC clustering at around E13.5 to E14, followed by the appearance of bonafide NEBs, which contained mature PNECs at around E15.5 to E16 (25). In human fetal lungs PNEC clustering was first observed at about 9 to 10 weeks of gestation (28).
Although one might imagine that the formation of NEBs is likely achieved through proliferation of nascent PNECs, a different mechanism has been shown to be at play. Sparse lineage tracing of early fetal Ascl1-positive PNECs in mice using a multi-color lineage reporter showed that NEBs contained either different colored cells or a single labeled cell and multiple unlabeled cells, arguing that the PNECs in NEBs are not clonal (25). Live cell imaging of fetal mouse lung tissue showed that NEBs are formed through the migration and subsequent aggregation of PNECs at airway branchpoints (25,29). This process appears to be regulated by Slit-Roundabout (ROBO) signaling, a pathway more classically associated with axonal guidance (30). PNECs express the ROBO receptor and the lungs of mice where the Slit-ROBO pathway has been disrupted by mutation of either the Slit ligands or the ROBO receptor itself, have fewer NEBs and more solitary PNECs than the lungs of wildtype mice (21).
Concomitant with NEB formation, the transcriptional events initiated by expression of Ascl1 culminate in the emergence of fully differentiated, functional PNECs. In particular, expression of Ascl1 in lung progenitors induces expression of the zinc finger transcription factor, INSM1. PNECs in mice carrying mutant alleles of Insm1 fail to express the mature PNEC markers, CGRP and UCHL1 (ubiquitin C-terminal hydrolase L1, a.k.a. PGP9.5). While the INSM1 targets that mediate the PNEC maturation process have not been delineated, INSM1 has been shown to directly repress the bHLH transcription factor and Notch target gene, Hes1 (Figure 2B) (31).
HES1 and other Notch signaling components play crucial roles in repressing the differentiation and specification of PNECs and, thereby, in mediating the NE versus non-NE cell fate choice. The lungs of mice in which Hes1 has been conditionally deleted in early lung progenitors have more ASCL1-positive cells and, in particular, fewer solitary PNECs, showing instead more and larger NEBs compared to the lungs of wildtype mice (29). The increased size of NEBs in Hes1-deficient lungs suggests a mechanism of cell fate specification through Notch-mediated lateral inhibition whereby Notch is activated in PNEC neighboring cells through binding of Notch receptors to the Notch ligands expressed on the surface of PNECs themselves. The activated Notch signaling in these PNEC neighboring cells induces expression of Hes1, which in turn represses the PNEC fate.
PNECs express the Notch ligands Dll1, Dll4, Jag1, and Jag2 shortly after their specification during lung development and the cells surrounding PNECs in NEBs express Notch receptors (32). Genetic loss of either all three Notch receptors or Dll1 and Dll4 Notch ligands in the developing mouse lung leads to a dramatic increase in the number and size of NEBs, phenocopying conditional deletion of Hes1 (32,33). In cultures of human airway cells derived from induced pluripotent stem cells, inhibition of Notch signaling leads to increased numbers of PNECs (34,35).
As we will discuss in other parts of this text, two other bHLH transcription factors, NEUROG3 and NEUROD1 have been shown to play central roles in the differentiation of EECs and pancreatic islet cells. In contrast, as of yet, there is little evidence that these transcription factors are decisive for PNEC specification or differentiation. To date, Neurog3 expression has not been described in PNECs. While Neurod1 expression has been observed in some PNECs in both fetal and adult mouse lung, a limited number of studies have investigated its specific role in the PNEC lineage (27,36). Neurod1-null mouse lungs from mice less than 2 weeks old showed decreased numbers of solitary PNECs and more NEBs compared to lungs from age-matched wild type mice. However, this difference normalized once mutant mice were 6 weeks old (36). As will be discussed later on in this text, NEUROD1 is a marker of a subtype of the high-grade lung NEN, small cell lung cancer (SCLC), suggesting it might also play a role in normal PNEC biology.
Reactive PNEC Proliferations: PNECs in the Response to Lung Injury
Multiple studies have shown that PNEC numbers and NEB size are altered in several human disease conditions. Increased numbers of PNECs have been observed in the lungs of patients with COPD, asthma, cystic fibrosis, and some forms of pneumonia. Other pathological conditions associated with increased PNECs include sudden infant death syndrome (SIDS), bronchopulmonary dysplasia (BPD), and congenital diaphragmatic hernias (CDH) (15). Studies in animal models and PNEC culture systems provide experimental evidence that PNECs respond to environmental stimuli by both proliferation and/or secretion of bioactive compounds.
The early observation that murine PNECs proliferate in response to a common form of experimental lung injury, naphthalene administration, and that this proliferation precedes epithelial lung regeneration, led to the hypothesis that PNECs are multipotent stem cells that aid in lung regeneration (37). Indeed, lineage tracing studies of PNECs following naphthalene induced lung injury showed that a rare subpopulation of PNECs, termed NEstem, can function as stem cells in this context (38). In response to naphthalene, NEstem cells proliferate and sometimes migrate to the site of injury where they dedifferentiate (lose NE identity) and take on other lung cell fates. The process of dedifferentiation and reprogramming was shown to be mediated by Notch signaling, recalling the role of this pathway in PNEC fate specification, and by EZH2 (38,39). Nonetheless, NEstem cells are not solely responsible for regenerating the lung after injury, as they were shown to contribute only to a small portion of the regenerated surface epithelium (38). Furthermore, genetic ablation of PNECs does not abrogate lung regeneration following naphthalene injury (23,39).
In thinking about PNECs as components of a diffuse hormonal system, two questions arise from the studies of PNECs in the context of naphthalene lung injury. The first is, how do PNECs detect injury? Club cells, which express cytochrome P450 2F2 (Cyp2f2), metabolize naphthalene to a toxic metabolite and the accumulation of this toxic metabolite leads to cell death specifically in these cells (40). Given that PNECs proliferate at time points shortly after peak Club cell injury, it is likely that they are responding to the Club cell injury and not to the naphthalene itself. Consistent with this hypothesis, selective ablation of Club cells using genetic ablation techniques also resulted in PNEC proliferation (41). Nonetheless, injury associated signals that are specific to PNECs and their responses have not been identified.
The second question that arises is, besides functioning as stem cells, do PNECs have an endocrine or paracrine/autocrine function in the context of lung injury? Although this question has not been explored in the naphthalene injury model, evidence from other model systems and from human diseases suggest that PNECs respond to some forms of lung injury or disease through the secretion of bioactive compounds. Cigarette smoke, a common culprit of lung injury, provides a good example. Bronchoalveolar lavage (BAL) fluid from smokers has increased levels of peptides secreted by PNECs, implicating these cells in the cellular response to cigarette smoke in humans (42). The PNEC-secreted bioactive compounds associated with this response include GABA and GRP, and both of these molecules have been implicated in inflammation and inflammation-associated processes (42,43). It is likely that PNEC proliferation is also involved in the response to cigarette smoke and its primary component, nicotine. Increased PNECs have been observed in rats exposed to cigarette smoke pre- and postnatally and in rhesus monkeys exposed to nicotine prenatally (44,45).
Pointing to a critical role for PNECs in oxygen sensing in the lung, hypoxia and hypoxia-mimicking genetic modifications have been shown to result in higher numbers of PNECs in mice, rats, rabbits, and guinea pigs (22,46–49). Shortly after showing that the distinct dense cored vesicles of PNECs carried serotonin, Lauweryns and Cokelaere went on to show that this serotonin was secreted by PNECs upon exposure to hypoxia (14,49). This finding was further refined and shown to be dependent on changes in intracellular Ca2+ concentrations using cultured rabbit and hamster lung slices (50,51). Serotonin release by PNECs is likely a physiologically relevant functional response to hypoxia as serotonin has been shown to induce vasoconstriction of pulmonary arteries (52).
Increased expression in PNECs of the neuropeptide, CGRP, has also been linked to hypoxia (46,53). CGRP has been implicated in promoting alveolar regeneration and in mediating immune cell responses in the lung (21,54). Importantly, results from a study by Shivaraju et al. linked the expression of CGRP by PNECs to the hypoxia-induced regenerative response of epithelial cells in the trachea. When the authors ablated PNECs and exposed mice to hypoxia, they observed a defective regenerative response that could be rescued by intranasal administration of CGRP (46).
PNECs appear to also respond to hyperoxia-induced lung injury. Patients with BPD, a chronic lung disease associated with oxygen supplementation of premature infants, have increased numbers of GRP-expressing PNECs (55). In a baboon model of BPD, some of the lung defects associated with the disease could be prevented by treatment of the animals with a GRP blocking antibody, demonstrating that GRP is directly linked to the disease phenotype (56). GRP also plays a role in the lung’s response to viral pneumonia and in the fibrotic response to radiation therapy (57,58).
There is clear evidence for a close interplay between PNECs and immune cells. In particular, the effector molecules secreted by PNECs can recruit and activate different populations of immune cells. In one of the first studies to show this, researchers developed a mouse model of CDH, a birth defect that results in pulmonary hypoplasia and pulmonary hypertension (21). CDH is associated with both a heightened immune response and increased numbers of PNECs (59). To study CDH, since point mutations in SLIT and ROBO genes are associated with the disease, Branchfield et al. generated mice with lung-specific deletions of the roundabout receptors, Robo1 and Robo2. When Robo1 and Robo2 were deleted in the entire lung epithelium the authors noted elevated immune cell infiltration in the lung, thus mimicking one of the features of CDH. When Robo1 and Robo2 were deleted only in PNECs, the mice displayed the same phenotype, directly linking the defect to PNECs. Interestingly, ROBO1- and ROBO2-deficient PNECs have increased levels of CGRP and knockout of the gene encoding CGRP partly reversed the immune and lung phenotypes of mice deficient for ROBO1 and ROBO2 in the lung epithelium.
A potential immune regulatory role for PNECs is also suggested by the observation that PNEC numbers are elevated in patients with asthma and that more chromogranin A-positive PNECs are seen in guinea pigs after allergen sensitization and challenge (60). Mice deficient of PNECs due to deletion of Ascl1 in the lung epithelium, show a dampened response to allergen challenge -- reduced goblet cell hyperplasia and reduced immune cell infiltration -- and this was tied directly to reduced levels of PNEC-derived GABA and CGRP, respectively (61).
Diseases of Primary PNEC Hyperplasia: NEHI and DIPNECH
Up to now we have highlighted instances of increased PNEC number or NEB size that appear to be consequent to or at least associated with some forms of acute or underlying lung injury. These examples are instructive in that they point to a role for PNECs and the molecules they secrete in mediating the response to external stimuli and injury in the lung. Save for lung NENs, which will be discussed in further detail later in this text, there are two notable clinical instances of primary -- as opposed to reactive -- PNEC proliferation that have no known etiology and are not associated with common pathogenic triggers: neuroendocrine cell hyperplasia of infancy (NEHI) and diffuse idiopathic neuroendocrine hyperplasia (DIPNECH).
NEHI is a rare pediatric lung disease consisting histologically of hyperplastic GRP-positive and serotonin-positive PNECs in the distal lung epithelium of otherwise normal lung tissue. Symptoms are usually first noted between 6 to 8 months of life and include tachypnea, retractions, crackles and hypoxemia (62). In some cases, patients with NEHI show an inconspicuous, patchy pattern of inflammation or fibrosis, generally assumed to be a consequence of the increased PNEC numbers rather than its cause (63). Interestingly, despite increased PNEC numbers in the lungs of patients with NEHI, from a study on a small patient cohort (5 patients), it appeared that PNECs were not actively proliferating in the lungs of these patients as no Ki67 and GRP double positivity was observed (63). Unfortunately, treatment for patients with NEHI are currently limited to supportive oxygen supplementation and, in some cases, additional nutritional support. The majority of NEHI patients show gradual improvement of symptoms and the disease is not associated with mortality. Nonetheless, recent reports show that some patients experience abnormal lung function persisting into adulthood (62,64,65). While the etiology of this disease remains unknown, there are indications of a genetic basis for the disease. One study identified four families with multiple members diagnosed with NEHI and showing an autosomal dominant pattern of inheritance (66). Another study identified a heterozygous mutation in the NKX2.1 gene in members of a family with a history of childhood lung disease consistent with NEHI (67).
DIPNECH is a rare syndrome with adult onset consisting histologically of increased PNECs in the small bronchi and bronchioles and confined to the basement membrane, appearing as scattered PNECs, small nodules, or a linear proliferation of PNECs (68). These features are often seen in concomitance with what are referred to as tumorlets, PNEC proliferations that extend beyond the basement membrane but are less than 5 mm in diameter (69). Other histological features include fibrosis, chronic inflammatory cell infiltrate, and constrictive obliterative bronchiolitis. The majority of patients with DIPNECH are women and the disease is not associated with smoking or other lung diseases. Patients diagnosed with DIPNECH often present with symptoms including cough, exertional dyspnea and an obstructive or mixed obstructive/restrictive defect on pulmonary function test. A small number of patients with DIPNECH are diagnosed due to incidental findings (69).
DIPNECH was first recognized and formally defined in 1992 by Aguayo et al., who described the symptoms and histological features of 6 DIPNECH patients (42). While DIPNECH is considered a disease of primary rather than reactive PNEC proliferation, cases associated with parathyroid gland hyperplasia, acromegaly and pituitary adenoma, multiple endocrine neoplasia type I syndrome, and pulmonary adenocarcinoma have been reported (70–72). The World Health Organization (WHO) classifies DIPNECH as a preinvasive, possibly preneoplastic condition (73). Most patients with DIPNECH have multiple PNEC nodules, sometimes including both tumorlets and frank low grade lung NET (carcinoid) tumors (70). In contrast to NEHI where Ki67-positive PNECs were not observed, the PNEC proliferations in DIPNECH patients contain some Ki67-positive cells (63,74). While patients with DIPNECH most often follow a clinical course showing stability or slowly progressing functional decline, a small subset of patients have rapidly progressive disease including progression to respiratory failure or metastatic carcinoid tumors (70,75). To date, there is no standard of care for DIPNECH and the most effective treatment strategy for patients with DIPNECH are somatostatin analogues (SSAs), which have shown effectiveness in improving symptoms of cough and dyspnea in some patients (76–78). Considering that it has been well-established that SSAs inhibit the secretion of bioactive compounds from gastrointestinal NETs, the effectiveness of SSAs in treating cough and dyspnea in patients with DIPNECH suggests these symptoms are caused by the secretion of bioactive compounds by DIPNECH PNECs (79).
Lung NENs
Lung NENs account for 20-25% of all lung cancers and for 25-30% of NENs from all tissue sites (80,81). As is the case for NENs in general, lung NENs comprise both low grade, well-differentiated NETs and high grade, poorly differentiated NECs. Lung NENs can thus be subdivided into the high-grade carcinomas, small cell lung cancer (SCLC) and large cell neuroendocrine carcinomas (LCNEC), and the low-grade tumors, atypical carcinoids (AC), classified as intermediate grade, and typical carcinoids (TC), classified as low grade.
SMALL CELL LUNG CANCER (SCLC)
The most common lung NEN, SCLC, accounts for 79% of all lung NENs and 30% of all lung cancers and is also the best studied among lung NENs (82). Consistent with its classification as NEC, SCLC is a highly aggressive tumor with a high rate of metastasis and a 10-year survival rate of only 1-2% (83). Among patients with SCLC, 97% have a history of smoking (18). While rare, patients with SCLC sometimes experience paraneoplastic endocrine syndromes, most commonly a syndrome of inappropriate antidiuretic hormone (SIADH) and ectopic Cushing’s syndrome (84). Studies of SCLC biology have been aided by a collection of tumor-derived cell lines, several patient-derived xenograft (PDX) models, and genetically engineered mouse models (GEMMs) of the disease (85–87). These preclinical model systems have allowed scientists to address questions in two key areas: the cell of origin of SCLC and molecular signatures predictive of therapeutic vulnerabilities.
Genetically, SCLC is a relatively homogeneous disease -- RB1 and TP53 are both almost universally lost in patient tumors (88). Conditional simultaneous genetic deletion of Rb1 and p53 in the mouse epithelium results in tumors that recapitulate many of the key features of the human disease at both the histological and molecular levels (87,89–91). Targeting the deletion of Rb1 and p53 to specific epithelial cell types in the lung provided definitive evidence that Cgrp-expressing PNECs are a cell of origin for tumors in this model (23,92). However, PNECs are not the only epithelial lung cell type that can be a cell of origin for mouse SCLC. A separate study showed that an, as of yet, unidentified CGRP-negative cell that is also negative for the canonical markers of two other common lung epithelial cell types gives rise to mouse SCLC lesions that are molecularly distinct from those initiated in CGRP-positive PNECs (93).
Genomic analysis of human and mouse SCLC primary tumors and cell lines has revealed commonly mutated genes and pathways, most notably loss of PTEN, NOTCH, and histone modification genes, and amplification of MYC family oncogenes (88,90,91). Several studies focused on metastasis in mouse SCLC have highlighted the role of NFIB in driving progression of some of these tumors, and data from human patients with SCLC support the clinical relevance of these findings (94–96). These studies, in combination with preclinical testing in mouse models and cell lines have suggested some degree of patient stratification. In particular, high expression of MYC is associated with tumor sensitivity to Aurora Kinase inhibitors (97).
The standard treatment regimen for patients with SCLC is a combination therapy of a platinum agent combined with etoposide (82). Despite a clinical response to these therapies in the majority of patients, almost all patients will then experience tumor recurrence (83). The analysis of the transcriptomes of both mouse and human SCLC tumors and cell lines has identified 4 molecular subtypes of SCLC, defined by their expression (or lack of expression) of 3 lineage-specific transcription factors: ASCL1 high, NEUROD1 high, POU2F3 high, and a fourth subtype that has low expression of NE transcription factors and has been proposed to be defined by expression of YAP1. A more recent study suggests a classification in which this fourth subtype is defined by expression of immune checkpoint genes and human leukocyte antigens (98,99). It has been hypothesized that the cell of origin for the POU2F3 high subtype of SCLC might be the pulmonary tuft cell, another chemosensory cell type in the lung distinct from PNECs (100,101).
Importantly, several studies using preclinical models of SCLC suggest that these molecular classifications can be used to stratify patients according to potential therapeutic vulnerabilities (102). Adding complexity to this schema, single cell RNAseq studies of mouse SCLC and of xenografts derived from circulating tumor cells from SCLC patients suggest that different molecular subtypes might represent different stages of progression where tumors begin in an ASCL1-high state and progress towards a non-NE state and that individual tumors might comprise cells belonging to different subtypes (101,103). Several studies have also shown other forms of intratumor heterogeneity in SCLC that have implications for patient therapy (104–106). Other new therapies suggested for SCLC include tricyclic antidepressants, therapies that target specific metabolic vulnerabilities, and therapies targeting the GNAS/ PKA/PP2A signaling axis (107–109).
LARGE CELL NEUROENDOCRINE CARCINOMA (LCNEC)
Pulmonary LCNEC is less common than SCLC, accounting for 16% of all lung NENs (82). Like SCLC, pulmonary LCNECs are highly metastatic and are associated with smoking history and with an overall 5-year survival rate ranging from 15% to 25% (110). In contrast to SCLC, however, there are relatively few preclinical models for LCNEC and we know much less about the basic biology of this disease. This might partly explain why guidelines for treating patients with LCNEC are rather rudimentary (82,111).
Pathohistological analysis of tumors from GEMMs of SCLC found that a portion of the mouse tumors in these models had a histological pattern consistent with LCNEC. While these LCNEC tumors only accounted for 10% of the tumors from the GEMM in which only Rb1 and p53 were conditionally deleted in the lung epithelium, they were much more prominent in the GEMM in which Rb1, p53, and Pten were conditionally deleted specifically in CGRP-expressing PNECs (87). A different GEMM, based on loss of Rb1 and expression of mutant p53 alleles, also develops both SCLC and LCNEC mouse tumors (112). These GEMMs have, thus far, not been used to explore the biology specifically of LCNEC and doing so might present some technical challenges. Recently, the first GEMM specifically for LCNEC was reported. In this model, Rb1, p53, Pten, and Rbl1 were simultaneously deleted in the mouse lung epithelium, resulting in a tumor spectrum consisting primarily of LCNEC and low-grade NETs (113).
The majority of insights into LCNEC have been provided by molecular analysis of primary patient tumor samples. The most comprehensive analysis, consisting of whole exome sequencing of 60 LCNEC tumors and RNA-sequencing expression analysis of 69 LCNEC tumors, highlighted the existence of two major molecular subtypes of LCNEC (114). Type I LCNECs had a higher rate of alterations in TP53 and STK11/KEAP1 and an NE expression profile defined by high expression of ASCL1 and DLL3 and low expression of NOTCH. Type II LCNECs had frequent mutations in RB1and TP53, therefore resembling SCLC at the genomic level. The expression profile of type II LCNECs, however, was distinct from SCLC and instead was defined as NE low, with low expression of ASCL1 and DLL3 but high expression of NOTCH.
The description of these two molecular subtypes for LCNEC highlights a clinical conundrum relating to the treatment of patients with LCNEC: should they be treated with SCLC chemotherapy regimens or with chemotherapy regimens for non-NE non-small cell lung cancer (NSCLC) (111)? The report of an SCLC-like subtype of LCNEC (type I) and a NSCLC-like subtype of LCNEC (type II), might suggest a way to stratify patients for different chemotherapy regimens. In line with this idea, a retrospective analysis of LCNEC cases found that patients whose tumors either had wildtype RB1 or showed expression of RB1 protein had a better outcome when treated with a NSCLC chemotherapy regimen as opposed to a SCLC chemotherapy regimen (115).
Other therapies beyond traditional chemotherapy regimens are also being explored for patients with LCNEC. One example that relates to patient stratification according to LCNEC subtype, which was defined in part by differential patterns of DLL3 expression, involves therapeutic strategies that use DLL3 to target tumor cells. Given that DLL3 is also expressed by some SCLC tumors, this also represents a potential therapeutic opportunity in SCLC. Although a DLL3-antibody conjugated to the DNA-damaging pyrrolobenzodiazepine dimer toxin did not provide a survival benefit in 2 phase 3 clinical trials, other DLL3 targeting approaches are being developed (116). In addition, several studies have uncovered potentially targetable molecular alterations in some LCNEC tumors, including activating EGFR mutations, FGFR1 amplifications, activating BRAF mutations, ALK rearrangements, and mutations affecting BDNF/TrkB signaling (114,117–119). Given that the majority of these targetable mutations have been identified in LCNEC tumors with wildtype RB1, the question remains as to how best to treat patients with RB1 mutant LCNEC (120).
LUNG NETs: TYPICAL AND ATYPICAL CARCINOIDS
Lung NETs comprise low grade typical carcinoids (TC) and intermediate grade atypical carcinoids (AC), accounting for 5% and 0.5% of all lung NENs, respectively (82). TC and AC tend to present in younger patients than LCNEC and SCLC, and the majority of patients are women and non-smokers (121). Although the majority of lung NETs are sporadic and non-functional, a small percentage of patients with Lung NETs, 5% of TC and < 2% of AC, present with paraneoplastic syndromes including those associated with adrenocorticotropic hormone (ACTH), growth hormone releasing hormone (GHRH), histamine, and serotonin (122,123). Some of these are more commonly associated with metastatic lesions of TC or AC (123). Approximately 5% of lung NETs are associated with the familial cancer syndrome caused by germline mutations in multiple endocrine neoplasia gene type I (MEN1). Interestingly, 5% to 10% of lung NETs are also associated with tumor multiplicity, a feature which might suggest a connection with either an unappreciated familial predisposition syndrome or with premalignant conditions such as DIPNECH (80,121).
The overall 10-year survival rates for stage I TC and AC are comparable ranging from 98% to 91%, respectively. In the case of stage IV tumors, 10-year survival for TC patients is 49% but is only 18% for patients with AC (124). A distinguishing feature of TC and AC is their relatively slow growth. Indeed, the pathological criteria for diagnosing carcinoids are the number of mitoses per mm2 and the presence of absence of necrosis: < 2 mitoses per mm2 and no necrosis for a diagnosis of TC, 2 to 10 mitoses per mm2 and demonstration of necrosis for a diagnosis of AC (82). Morphologically, carcinoids typically contain small cells that show nested, rosette, and trabecular growth patterns with peripheral palisading (125).
Complete surgical resection is the most common treatment for patients with TC and AC, and for the majority of these patients’ surgery is associated with a favorable survival prognosis. Unfortunately, however, a fraction of carcinoid tumors metastasizes, and tumor recurrence (even after apparent curative resection) has been reported in 1 to 6% and 14 to 29% of patients with TC and AC, respectively. Due to a highly variable time to relapse for patients with recurrence (0.2 to 12 years), the recommended follow-up period is 15 years (121,126–129). The reported incidence for lymph node metastasis for TC and AC is variable with rates ranging from 12% to 17% for patients with TC and from 35% to 64% for patients with atypical carcinoid (80,130). The incidence of distant metastases for both TC and AC is 3% and 21%, respectively (129,131).
The incidence of tumor recurrence and metastasis calls attention to a clinical need for systemic treatment options for patients with TC and AC tumors that are unresectable, as well as for the need for effective adjuvant therapy options that can be offered to patients after surgery. Unfortunately, standard chemotherapy and radiotherapy regimens have proven to be mostly ineffective in this patient population (80). The only treatment option shown to improve progression-free survival in patients with advanced and progressive TC and AC is the mTOR inhibitor everolimus (132).
Other therapeutic options for patients with TC and AC that are not currently considered standard of care due to limited clinical trials, include somatostatin (SST) analogues and peptide receptor radionuclide therapy (PRRT), and temozolomide with or without capecitabine. Given that pulmonary carcinoids can express SST receptors, patients with these tumors can be potentially considered for palliative treatment with unlabeled or radiolabeled SST analogues.
The lack of clarity regarding standard of care for patients with unresectable, metastatic, or recurrent TC and AC, points to an unmet need for not only new and effective systemic treatment strategies for these patients, but also for clear patient stratification criteria for predicting the probability of a response of a given patient tumor to specific therapeutic options. Furthermore, given the broad range of tumor malignancy for TC and AC, biomarkers that can predict the potential for tumor progression and metastasis or recurrence are also needed. Efforts to address these clinical needs have been hindered by both difficulties in performing molecular characterization of these tumors and by a dearth of preclinical models representative of the disease. Only a handful of cell lines exist for TC and AC and only one GEMM for TC and AC has been reported (133,134).
In contrast to SCLC and LCNEC, pulmonary carcinoids have a low tumor mutational burden, have very few recurrent or characteristic mutations, and rarely contain “driver” mutations in known oncogenes. The most commonly mutated gene in pulmonary carcinoids is MEN1, and up to 5 to 13% of patients with germline mutations of this gene are diagnosed with pulmonary carcinoids (135–137). The most commonly mutated class of genes in pulmonary carcinoids are chromatin remodeling genes, a category that includes MEN1, PSIP1, and ARID1A. Though prevalent in SCLC and LCNEC, mutations in RB1 and TP53 are rare in pulmonary carcinoids (135,136). Recurrent copy number alterations have also been identified in pulmonary carcinoids, including in genes that would imply targetable therapeutic vulnerabilities such as, EGFR, MET, PDGFRB, AKT1/PKB, PIK3CA, FRAP1, RICTOR, KRAS, and SRC (136,138).
Transcriptional and methylation analysis of primary pulmonary carcinoids has also revealed distinct subclasses of these tumors. Using multi-omics factor analysis (MOFA), Alcala et al. identified 3 molecular clusters, termed A1, A2, and B (139). While most of the tumors in clusters A1 and A2 were TC, tumors in cluster B were primarily classified as ACs. Tumors in cluster B had high expression of ANGPTL3 and ERBB4, were enriched for mutations in MEN1, and were associated with a worse overall survival. Consistent with a worse prognosis for patients with tumors in cluster B, tumors in this cluster also showed universal downregulation of the orthopedia homeobox protein gene, OTP, whose expression has previously been associated with an improved prognosis in patients with pulmonary carcinoids (126). A separate study performed a similar multi-omic analysis of an independent set of pulmonary carcinoids and also identified 3 molecular subtypes that they termed LC1, LC2, and LC3 (140). The concordance between the molecular subtypes identified in these two studies was shown through integration of the two datasets, further validating the use of these molecular classifications for pulmonary carcinoids (141).
The molecular analysis of pulmonary carcinoids has provided evidence that supports the idea that a fraction of lung NENs may actually fall into a category that lies between G2 ACs and G3 NECs in terms of malignancy. While such a category is recognized in GEP-NENs and is termed well-differentiated G3 NET, its existence has only recently been suggested for lung NENs (142). The study by Alcala et al. identified a subgroup of ACs, termed “supra-carcinoids,” that showed the morphologic characteristics of pulmonary carcinoids, but whose transcriptional profile was closer to that of LCNECs (139). In their analysis of the transcriptional profiles of a series of LCNECs and ACs, Simbolo et al. identified 3 molecular clusters, C1, enriched for LCNECs, C3 enriched for ACs, and C2, which was mixed in terms of number of ACs and LCNECs and which showed intermediate molecular features (143). Finally, an earlier study by Rektman et al. had identified 2 examples of what they referred to as “carcinoid-like” LCNEC tumors -- tumors that showed a clear carcinoid-like morphology and a molecular profile consistent with ACs (low tumor mutational burden and mutation in MEN1) but that had been classified as LCNEC due to a high proliferation rate (118).
The supra-carcinoids in the Alcala et al. study showed a higher expression of MKI67 than other carcinoids in the series, supporting an idea that has been purported in the literature concerning a potential role for percent Ki67 positivity in identifying pulmonary carcinoids more likely to be associated with a poor prognosis (144–146). Typically, ACs show a Ki67 positivity rate of less than 20%. However, some tumors diagnosed as AC show rates between 20 and 50% (147,148). Likewise, as indicated by the “carcinoid-like” LCNEC tumors in the Rekhtman et al. study, some tumors that would otherwise be considered ACs, are diagnosed instead as LCNEC due to having a high proliferation rate (118,149). Furthermore, the comparison of proliferation rates between matched primary stage IV pulmonary carcinoids and metastases indicated an increased proliferation rate in 35% of the metastases, suggesting increased proliferation as a feature of progression (148). This idea is further supported by the observation that Ki67 positivity was heterogeneous in the analyzed tumors with some regions of the tumors showing hot-spots of increased proliferation compared to the rest of the tumor. Beyond Ki67 positivity, a list of defining features of supra-carcinoids or borderline pulmonary carcinoids/neuroendocrine carcinomas has yet to be established.
ENDOCRINE CELLS IN THE GASTROENTEROPANCREATIC TRACT
Together, the organs connected throughout the mouth to the anus are known as the gastrointestinal (GI) tract, and when the pancreas is included, these organs are collectively referred to as the gastroenteropancreatic (GEP) tract. Throughout the GEP tract endocrine cells can be found as either solitary cells, as is the case in the GI tract, or as innervated clusters, as is the case in the pancreas.
Throughout the gastrointestinal (GI) tract the solitary endocrine cells, which have a slender, elongated shape, are referred to as enteroendocrine cells (EECs). This classification helps to distinguish them from endocrine cells of other organs e.g., lung and pancreas. Despite representing only 1% of the gut epithelial cells, the large size of the intestinal epithelium makes it the body’s largest endocrine organ (150,151).
Compared to the slender EECs of the GI tract, the pancreatic endocrine cells, dispersed as clusters (known as islets of Langerhans) throughout the organ, have a more pyramidal or round-oval shaped appearance. An adult human has millions of islets, which collectively correspond to roughly 2% of the pancreatic epithelium (152,153). These islets are highly vascularized, a feature that enables pancreatic hormones to travel via the bloodstream to reach their target organs. In fact, the pancreatic hormones act both locally and systemically, eliciting responses throughout the body, consequently affecting the overall metabolic state of the organism. The sections below will provide an overview of the endocrine cells of the GEP tract as components of both the diffuse neuroendocrine system and the body’s diffuse hormonal systems.
GASTRIC ENDOCRINE CELLS
The first major organ of the GI tract is the stomach, which, in humans, can be divided into 4 functionally distinct compartments. From proximal to distal; the cardia is the connective region between the esophagus and the stomach, the fundus stores undigested food and gases, the corpus is the largest compartment and performs the digestive action of the stomach, and, finally, the pylorus regulates gastric emptying (Figure 3A) (154,155).
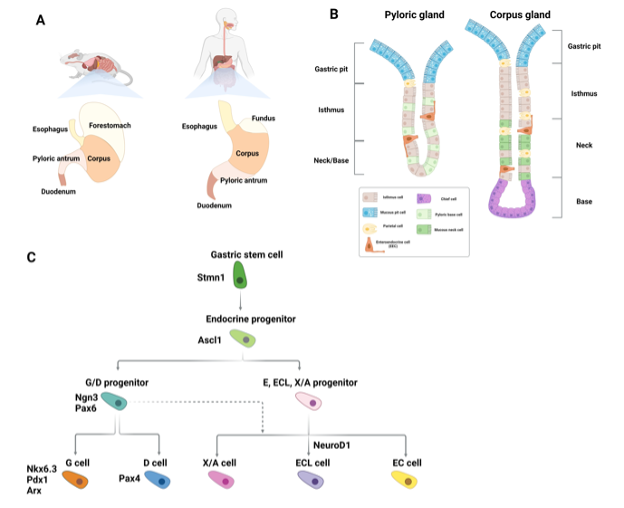
Figure 3. Gastric enteroendocrine cells. (A) Schematic showing the anatomical differences between the murine and human stomach (B) Schematic depicting epithelial cell types found in the gastric pylorus and corpus glands. Solitary gastric enteroendocrine cells are shown in orange. (C) Diagram of signaling and transcription factor interactions that regulate gastric enteroendocrine cell differentiation.
Histologically, the stomach comprises tubular-shaped mucosal invaginations containing a pit region of primarily surface mucous cells, and a gland region. The latter is further subdivided into the isthmus, neck, and base (Figure 3B). The primary differentiated cell types of the stomach are: mucus-producing pit cells, chief cells, which secrete digestive enzymes, acid-secreting parietal cells, gastric tuft cells, whose function is ill defined, and gastric EECs. These cells are continuously formed throughout life, albeit at different rates, by progenitor cells located in the isthmus of the gland region (156,157). With the exception of the cardia, which primarily contains pit cells and scattered parietal cells, gastric EECs can be found in all of the compartments of the stomach. In the corpus and fundus EECs are located in the lower third of the glands. EECs in the pylorus are located in the neck region (158).
Gastric EECs are divided into the following 5 main subtypes defined by their predominantly expressed hormone: G cells (gastrin), D cells (somatostatin), enterochromaffin (EC) (serotonin), EC-like (ECL) cells (histamine), and X/A cells (ghrelin) (Figure 3C) (159). While some gastric EEC subtypes overlap with those found in the intestine, comparison of duodenal EECs and gastric EECs by single cell RNA sequencing (scRNA-seq) suggested a distinct gastric EEC expression profile. These differences are most likely reflective of the tissue specific microenvironment of these EECs, the stimuli they are exposed to, and their different functions (160).
As will be outlined in subsequent parts of this text, differentiation of intestinal EECs requires expression of the master bHLH transcription factor, NEUROG3. While NEUROG3 is important for gastric EECs, its role appears to be EEC subtype specific. Most gastric EECs are also dependent on ASCL1, the same transcription factor that initiates PNEC specification. Studies from two different groups, which independently generated Neurog3 null mice showed that while these mice lacked D-cells and G-cells and had decreased numbers of EC cells, ECL and X/A cells were unaffected (161,162). Ascl1 null mice displayed a similar but not identical phenotype, showing lack of D, G and EC cells and severely decreased numbers of X/A cells (163). ECL cells were not examined in Ascl1 null mice. Thus, some gastric EECs, such as D- and G- cells are dependent on both NEUROG3 and ASCL1, while others, such as X/A cells, are dependent on ASCL1 but not NEUROG3. EC cells appear to be entirely dependent on ASCL1, and only partially dependent on NEUROG3 (163). Ascl1 is not expressed in intestinal EECs from the mouse. Nonetheless, scRNA-seq of human intestinal EECs identified expression of ASCL1, suggesting a role for this transcription factor not only in gastric EECs but also in human intestinal EECs (164).
The EEC hormone most clearly associated with gastric function is gastrin, secreted by G-cells located in the pyloric compartment of the stomach. These cells are also found in the duodenum, but their function has been best studied in the stomach (160,164). Gastrin secreted into the bloodstream by G-cells binds to its receptor expressed by ECL cells in the corpus thereby stimulating them to secrete histamine, which, in turn, stimulates neighboring parietal cells to secrete gastric acid. Given that parietal cells themselves also express the gastrin receptor, gastrin release by G-cells can also directly stimulate parietal cells to secrete gastric acid (165–167). The other hormones produced by the gastric EECs are also secreted by other gastroenteropancreatic endocrine cells (GEP-ECs) and will be discussed in later sections.
INTESTINAL AND COLONIC ENDOCRINE CELLS
Architecture and Cell Types of the Intestine
The gut can be divided into the small and large intestine (also known as the colon). The two primary functions performed by the small intestinal epithelium are 1) to form a barrier against the continuous chemical and mechanical insults induced by the undigested food, microorganisms, and toxins present in the intestinal lumen, and 2) to absorb nutrients from ingested food (150). This latter process occurs with an exceptionally high efficiency made possible by the large surface area generated by the intestinal epithelium’s folded structure. Protrusions known as villi contain differentiated non-mitotic cells, while invaginations known as crypts contain proliferative, self-renewing stem cells and their epithelial niche cells, Paneth cells. The colon lacks villi and its primary function is water absorption and movement of the stool. The continuous damage experienced by the intestinal epithelium necessitates a high cellular turnover to maintain organ function. The intestinal stem cells, marked by the expression of leucine-rich G-protein-coupled receptor 5 (LGR5), continuously replace lost cells via rapid division which regenerates the epithelium within 4-5 days (150).
The differentiated cells that originate from the LGR5+ stem cells can be divided into two main functional categories, absorptive (enterocytes and microfold cells) and secretory (EECs, goblet, Paneth, and tuft cells) (Figure 4A). Intestinal EECs secrete hormones in response to stimuli such as nutrients from digested food and metabolites produced by the gut microbiota (168,169). The stimuli that EECs respond to can be both mechanical and chemical, and the hormones they produce are secreted both locally and into the bloodstream, thereby allowing them to act not just locally but also systemically. Gut hormones regulate important functions such as digestion, nutrient absorption, appetite, and gastric as well as gut motility (170).
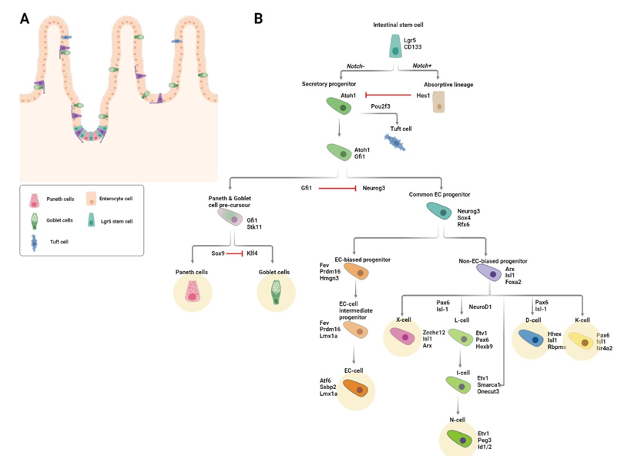
Figure 4. Intestinal enteroendocrine lineage specification. (A) Schematic of the intestinal epithelium. Solitary enteroendocrine cells (EECs) are depicted in purple. (B) Diagram of signaling and transcription factor interactions that regulate intestinal enteroendocrine cell differentiation. Differentiated EEC subtypes are highlighted with yellow circles.
Factors Regulating Commitment to the Secretory Lineage
The rapid division of LGR5-positive stem cells gives rise to progenitor cells, the majority of which differentiate as they migrate upwards along the crypt-villus axis. The following section describes the differentiation of intestinal EECs and highlights some of the essential regulatory factors and signaling pathways that direct this process.
NOTCH SIGNALING
The first step in becoming an EEC is commitment to the secretory lineage, a process that is initiated by the transcription factor, ATOH1 (Protein atonal homolog 1). At the crypt bottom, active Notch signaling prevents the differentiation of stem and progenitor cells. Cell-cell contact between Notch ligand (Dll1 and Dll4) expressing Paneth cells and stem/progenitor cells results in the expression of the Notch target gene, HES1, which in turn represses the secretory cell fate by repressing ATOH1 (171,172). Hence, commitment to the secretory lineage requires inactivation of Notch signaling.
Inactivation of Notch signaling is concomitant with loss of contact with Paneth cells. Due to the high ratio of progenitor to Paneth cells in the crypt, not all progenitors can simultaneously be in touch with a Paneth cell and this results in stochastic loss of Paneth cell-stem cell contact. Additionally, as new progenitors are continuously generated by the stem cells in the crypt, older progenitors are pushed upward along the crypt-villus axis, causing them to lose contact with the Notch ligand-presenting Paneth cells. The resultant loss of active Notch in these cells enables expression of ATOH1 and commitment to the secretory lineage (173,174). Mice lacking Atoh1 do not have any secretory cells (173). In contrast, mice with null alleles of Hes1 have excessive numbers of secretory cells.
Factors Regulating Commitment to the EEC Lineage
Transient expression of NEUROG3 commits Atoh1-expressing secretory progenitors to the EEC fate. Whereas Neurog3 knockout mice completely lack EECs, overexpression of Neurog3 leads to increased numbers of EECs and decreased numbers of goblet cells (162,175,176). Homozygous NEUROG3 mutations have been identified in children with generalized malabsorption and reduced numbers of intestinal EECs (177). Downstream targets of NEUROG3 include other transcription factors important for EEC differentiation such as NEUROD1, PAX4/6, NKX2.2, INSM1, and PDX1 (162,178–181). NEUROG3 is also implicated in cell cycle control: Neurog3 expression in mouse pancreatic endocrine progenitors leads to upregulation of the cell cycle inhibitor, Cdkn1a, and consequent cell cycle exit (182). Consistent with the idea that cell cycle exit biases secretory progenitors to the EEC lineage, inhibition of either epidermal growth factor receptor (EGFR) or mitogen activated protein kinase (MAPK) signaling induced quiescence of intestinal stem cells in organoid culture. When this quiescence was reversed by reactivation of these pathways, the resulting organoids had an increased proportion of EECs (183).
Specification of the Different EEC Lineages
While most progenitors generated in the crypt immediately begin migrating upwards, those primed to become EECs remain in the crypt for anywhere between 48h and 60h (184). During this time, these cells become committed to one of several divergent differentiation trajectories, each of which results in a different EEC subtype. Prior to leaving the crypt, EEC-committed progenitors have already started to express and secrete their lineage-defining hormones. The time required to produce a specific hormone varies between the different EEC lineages and this may explain why some EEC-committed progenitors remain in the crypt longer than others (184).
Altogether, intestinal EECs produce more than 20 different hormones. The earliest classification of EECs was based on immunostainings and consisted of the following 8 EEC lineages as defined by the main hormone they were found to express: enterochromaffin (EC) cells that secrete serotonin (5-hydroxytryptamine, 5-HT), I cells that secrete cholecystokinin (CCK), K cells that secrete gastric inhibitory peptide (GIP), L cells that secrete glucagon-like peptide 1 (GLP-1), X cells that secrete ghrelin (GHRL), S cells that secrete secretin (SCT), D cells that secrete somatostatin (SST), and N cells that secrete neurotensin (NTS) (185).
At the time that this classification was first proposed, it was believed that EECs belonging to a given subtype predominantly expressed the hormone that defined that EEC subtype. Thus, for example, it was believed that EC cells only predominantly expressed serotonin, and L cells only predominantly expressed GLP-1. However, new techniques for performing hormone co-stainings using multiple antibodies, fluorescent hormone reporter mice, and transcriptome-based sequencing of EECs have all led to the observation that some EECs express multiple subtype-defining hormones (186–190). The question thus arose, did these multihormonal EECs represent previously unidentified and distinct EEC subtypes? Or were they simply cells caught in a transition state along the EEC subtype differentiation trajectory? The latter would suggest that EECs are capable of hormonal plasticity and are therefore capable of transitioning from expression of one lineage defining hormone to another.
RESOLVING LINEAGE IDENTITY AND HORMONE SWITCHING
One of the first lines of evidence that EECs might undergo hormone switching was the observation that, while serotonin-producing EC cells were rapidly labeled after a single pulse of radioactive thymidine, secretin-producing S cells were labeled much later and only after multiple injections of the isotope (191,192). Thus, it was concluded that serotonin-expressing cells but not secretin-expressing cells had the ability to self-renew and that secretin cells did not differentiate before reaching the villus. Based on this data, one could postulate that serotonin-expressing cells might become secretin-expressing cells once they reach the villus.
A lineage relationship between EECs localized in the crypt and EECs localized in the villus was first suggested in 1990 by Roth and Gordon based on an immunohistochemical study in which it was observed that cells expressing substance P (encoded by the Tac1 gene) but not secretin were found in the crypt, cells expressing both substance P and secretin were found in the bottom of the villus, and cells expressing secretin but not substance P were found exclusively at the top of the villus. The majority of substance P-expressing and secretin-expressing cells co-expressed serotonin. The authors thus concluded that these hormones were sequentially expressed along the crypt villus axis (186). The fact that substance P-expressing cells were labeled by the thymidine analogue, BrdU, faster than secretin-producing cells further supported this idea of sequential expression of substance P and secretin by the same EEC (193). Functional evidence for dynamic hormone-switching in EECs was provided first by cell ablation studies showing that ablation of one EEC subtype led to decreased numbers of other EEC subtypes (194). Subsequently the generation of a novel mouse Neurog3 reporter allele, Neurog3Chrono, enabled more definitive delineation of dynamic hormone-expression patterns of single EECs (184).
In the Neurog3Chrono mouse, two fluorescent reporter proteins, an unstable mNeonGreen and highly-stable tdTomato, are expressed concurrently with endogenous Neurog3. As a result, the ratio of red to green fluorescence of a given EEC provides real-time information about the age of that cell relative to when it expressed Neurog3 (184). ScRNAseq of EECs from Neurog3Chrono mice provided definitive evidence that many EECs switch the hormone they produce throughout the course of their lives and furthermore suggested a more simplified EEC subtype classification consisting of five mature EEC lineages. One of these five lineages was the one proposed by Roth and Gordon of substance P expressing cells that give rise to serotonin-expressing cells and then to secretin-expressing cells. This lineage was also confirmed independently by lineage tracing of Tac1-expressing cells in the mouse intestinal epithelium (195).
Two key observations from the Neurog3Chrono study substantiated a simplified EEC lineage classification. First, all EEC lineages except for SST-expressing D-cells began to express secretin upon entering the villus, thus rendering the S-cell lineage obsolete. Second, L-, I- and N- cells were shown to belong to a single lineage. Prior to this, observations from a mouse model of L-cell ablation had led the Schwartz lab to propose that L- and N- cells were part of the same lineage (194). The Neurog3Chrono study showed that, when located at the bottom of the crypt, L-cells secrete GLP-1 but, upon reaching the upper regions of the crypt, begin to express the I-cell defining hormone, CCK, and, finally, upon reaching the villus region, they begin to express the N-cell defining hormone, NTS. Thus, the EEC lineages or subtypes could be reduced to the following 5 main lineages: enterochromaffin (EC) cells that secrete Serotonin, K cells that secrete GIP, X cells that secrete GHRL, D cells that secrete SST, and LIN cells that secrete GLP-1, CCK and NTS (Figure 4B) (184).
ENTEROCHROMAFFIN (EC) CELLS
EC cells are the most prevalent EEC subtype and they can be found in all regions of the intestine (196,197). They are slender, triangularly-shaped cells that can have protrusions extending towards the luminal surface of the intestine (198,199). EC cells are defined by their expression of both serotonin and tryptophan hydroxylase 1 (TPH1), an enzyme that catalyzes the rate limiting step in the biosynthesis of serotonin (189). Serotonin in EC cells is stored in pleomorphic granules and is released in response to chemical and mechanical stimuli. Although commonly associated with brain development and regulation of mood and stress, 95% of the body’s serotonin is produced by the intestine where it regulates functions such as motility, fluid secretion, and vasodilation (200–202). The response of EC cells to different types of stimuli is mediated, in part, through their expression of various receptors, including the olfactory receptor, OLFR558, which acts as a sensor for microbial metabolites, and the transient receptor potential A1 (TRPA1), a receptor-operated ion channel that detects dietary irritants (203). A subset of EC cells expresses the mechanosensitive channel Piezo2, which converts mechanical forces to secretion of fluids and serotonin (204).
Given the important functions exerted by serotonin, it is not surprising that EC cells are implicated in several GI pathologies (202). Consistent with the observation that most SI-NETs express serotonin, intestinal EC cells are thought to be the cell of origin for these tumors. An early hint that SI-NETs might indeed arise from EC cells was provided by a Immunohistochemical study in which serial sections of an entire ileal SI-NET tumor were stained for several EEC markers, including serotonin. The authors observed aggregates of proliferating EC cells within crypts in close proximity to the tumor, which the authors speculated were indicative of where the tumor had originated (205). A later study made the same observation in a larger cohort of SI-NET samples from eight different patients, supporting the idea that aberrant proliferation of EC cells within the crypt is associated with SI-NET formation (206).
More recently, it has been suggested that the cell of origin of SI-NETs is not necessarily a fully differentiated EC cell, but rather an EC cell that expresses not only the EC cell markers, TPH1 and CHGA, but also markers of reserve stem cells (207). As we will discuss later in this text (see, EECs can act as reserve niche and stem cells), lineage tracing of both progenitor cells and of cells expressing mature EC cell markers in the mouse intestinal epithelium has shown that EC cells can adopt a stem cell fate upon injury (208). This observation suggests a plausible scenario whereby, if an EC cell were to acquire a genomic (or other) aberration capable of driving NET genesis, it could be long lived enough to indeed give rise to a tumor. Consistent with this hypothesis, Sei et al. identified human EC cells co-expressing the EC cell marker TPH1, and markers associated with both canonical and reserve stem cells, within crypt EC cell microtumors in tissue sections from patients with familial SI-NETs (209,210).
WHAT CAUSES HORMONE SWITCHING?
Growth factor gradients change from high WNT, high EGF, high NOTCH, and low BMP, at the crypt bottom, to increasingly high BMP, low WNT, low EGF, and low NOTCH along the villus. Thus, upon leaving the crypt, EEC progenitors are exposed to increasingly different signaling environments. It was therefore attractive to speculate that these signaling gradients could induce hormone switching in EECs.
Adult stem cell (ASC) derived mouse and human intestinal organoids lack mesenchymal cells and are therefore not exposed to growth factor gradients but instead experience a constant environment determined by the media composition (211). Consequently, the ASC-derived intestinal organoid system provides researchers with a controlled, in vitro setting in which the signaling environment that intestinal cells experience can be modulated. Under expansion conditions that mimic the crypt environment and are optimized to promote stem cell maintenance, the EECs in mouse small intestinal organoids display a crypt hormone profile. However, when BMP4 was added to the culture media to mimic the villus environment, the EECs in the organoids expressed Secretin suggesting they had taken on a villus-like profile (195). The molecular mechanisms governing the hormone switching from GLP-1 to CCK to NTS observed in the L-I-N lineage remain to be unraveled but are likely to similarly involve cellular signals that differ along the crypt-villus axis.
Non-Neoplastic EEC Hyperplasia
The previous sections described the formation of intestinal EECs. These cells and the hormones they secrete play a central role in regulating processes that are important for maintaining organismal function and energy homeostasis. It is therefore not surprising that EECs are implicated in a number of human disease conditions. Increased plasma level of EEC hormones and, in some cases, direct evidence of increased numbers of specific intestinal EEC subtypes have been reported in inflammatory bowel disease (IBD), irritable bowel syndrome (IBS), lymphocytic colitis, Celiac disease, H. pylori infection, and Giardia infection (212–215). A genome-wide association study (GWAS) identified a strong association between a single nucleotide polymorphism (SNP) in the promoter of the EEC transcription factor, PHOX2B, and Crohn’s Disease (CD), a form of IBD (216). The mechanism leading to intestinal EEC hyperplasia in these conditions is not clear, though there is some evidence that inflammatory cytokines are involved (213). Consistent with this idea, treatment of mice with IFN and TNF led to increased numbers of Chromogranin A-positive colonic EECs (217). Likewise, IL-13 has been linked to the response of EC cells to enteric parasite infection (218).
It is plausible that the observed changes in EEC numbers in these conditions are not necessarily or not only a result of the disease pathology, but are also mediators of the pathology itself. There is clear evidence for interplay between EECs, the immune system, sensory neurons, and commensal bacteria (168,219). Together with goblet cells, EECs have been shown to secrete the cytokine IL-17C in patients with IBD (220). EEC hormones have been shown to have immunomodulatory functions (219). Some EECs are directly innervated and one study showed that serotonin-expressing intestinal ECs form synapses with nerve fibers through which they can modulate nerve fiber activity (203). EECs express functional toll-like receptors (TLR) and can thereby interact with commensal bacteria by responding to the metabolites they produce (221). Furthermore, as EEC hormones are known to also act systemically, the consequences of disease associated alterations in their abundance or function are not limited to the GI tract. Most notably, GLP-1 and GIP, which amplify glucose stimulated insulin secretion, are less effective in patients with type 2 diabetes (T2D) and GLP-1 receptor agonists are currently used to treat T2D and obesity (185).
The most well-documented examples of non-neoplastic EEC hyperplasia are hyperplasias of gastric and duodenal EECs. These include: ECL cell hyperplasia and G-cell hyperplasia. ECL-cell hyperplasia is associated with chronic excessive gastrin production, hypergastrinemia, resulting most commonly from achlorhydria due to chronic atrophic gastritis (CAG), gastrin-producing tumors in Zollinger-Ellison syndrome (ZES), and long-term proton-pump inhibitor (PPI) treatment (222). Lineage tracing of gastrin/cholecystokinin-2 receptor (CCK2R)-expressing cells in mice showed that CCK2R-expressing ECL cells in the isthmus but not the base of the stomach proliferated in response to PPI-induced hypergastrinemia (223). ECL cell hyperplasia only rarely progresses to neoplastic gastric ECL NETs. G-cell hyperplasia is most commonly observed as a secondary change associated with CAG in patients with pernicious anemia. In rare cases, it has also been observed in patients with peptic ulcer disease in conjunction with decreased numbers of a different gastric EEC, the SST producing D-cell. G-cell hyperplastic lesions do sometimes progress to G-cell NETs, gastrinomas (222).
EECs CAN ACT AS A RESERVE NICHE AND STEM CELLS
The microenvironment in which LGR5-positive intestinal stem cells reside is known as the stem cell niche and includes Paneth cells. The stem cell niche presents the stem cells with cellular signals that prevent them from differentiating and help preserve their self-renewal capacity. Loss of Paneth cells or severe injury such as irradiation, chemotherapy treatment, or surgery can cause loss of intestinal stem cells. In these situations, several different epithelial cell populations have been shown to act as reserve stem cells (224–228). The first studies indicating that EECs could act as reserve stem cells followed the fate of secretory progenitors (224–228). As this progenitor population also contained progenitor cells fated to become Paneth or goblet cells, the contribution specifically of EECs could not be explicitly determined. A later study that used lineage tracing of a population of EEC committed secretory progenitors expressing markers of differentiated EECs showed that these cells can act as reserve stem cells, capable of both contributing to intestinal regeneration after irradiation and generating organoids in vitro (228).
Lineage tracing of mouse intestinal cells expressing either NEUROD1 or Tryptophan hydroxylase (TPH1; the rate limiting enzyme in Serotonin biosynthesis and considered an EC cell marker) showed that these cells, similar to the EEC committed secretory progenitors mentioned above, had the ability to contribute to homeostasis and to regeneration following irradiation induced injury (208). Given that the EEC-committed progenitors in the earlier study expressed Neurod1, and that a subset of these cells also expressed Tph1, it is unclear whether the Tph1 lineage-traced cells with regenerative capacity in this study were indeed mature differentiated EECs or the same multi-potent EEC committed secretory progenitors described before. More recently, it was shown that, upon genetic Paneth cell ablation, mature EECs replaced the ablated Paneth cells, serving as Notch-presenting niche cells for intestinal stem cells (229).
PANCREATIC ENDOCRINE CELLS
Located in the upper left abdomen behind the stomach, the adult pancreas, although being one anatomical entity, originates from two individual buds that arise from either side of the distal foregut endoderm during early embryonic development. As development progresses, these buds fuse to form the final glandular composite organ consisting of two compartments, one exocrine and one endocrine, which contain functionally and morphologically distinct cell types. Acinar cells secrete digestive enzymes which are transported to the duodenum by mucin-secreting ductal cells, and together these two cell types comprise the exocrine compartment. The endocrine compartment comprises 5 different pancreatic endocrine cell (pEC) types, each of which secretes a specific hormone: alpha (glucagon), beta (insulin), gamma/ PP (pancreatic polypeptide), delta (SST) and epsilon cells (ghrelin). As is the case for EEC-derived hormones, pancreatic hormones act both locally and systemically. Indeed, some pancreatic hormones are transported throughout the body via the bloodstream and instruct other organs to, among other things, release or store glucose from the blood. Hence, the pECs play a key role in nutrient metabolism, digestion, and glucose homeostasis (230).
Development and Differentiation of Pancreatic Endocrine Cells
In contrast to the GI tract where EECs are generated throughout the life of the organism, the pECs are almost exclusively generated during the fetal development of the organ (231). This process is orchestrated by an interplay of regulatory transcription factors. Whereas some of these transcription factors are expressed constitutively, others play a transient yet essential role in mediating pEC lineage commitment and differentiation (see table 4). Murine models have made invaluable contributions to our understanding of pEC generation. In rodents, pancreatic development is divided into 3 transition phases, each outlined below (Figure 5).
Table 4. NE Cell Lineage Transcription Factors
|
Transcription Factor
|
Associated NE cell types
|
Role (specifically in NE cells)
|
ASCL1
|
PNECs and gastric EECs
|
Lineage specification (26,27,163)
|
NEUROG3
|
GEP NE cells
|
Lineage specification (transiently expressed) (161,162,175,176,232,233)
|
NEUROD1
|
PNECs*, Intestinal EECs (L-I-N lineage), and pECs (beta cells)
|
Specification of some NE cell subtypes (not defined in lung, secretin-expressing cells and I-cells in the intestine, beta cells in the pancreas) (27,36,255,407,408)
|
ATOH1
(a.k.a. MATH1)
|
Intestinal EEC precursors
|
Specification of the intestinal secretory cell fate (precedes EEC lineage commitment) (173)
|
INSM1
|
PNECs and GEP EECs**
|
NE cell maturation (31,160,180)
|
GFI1
|
PNECs and Intestinal EECs
|
Maturation of CGRP+ PNECs (409); lineage specification (via inhibition of EEC fate in intestinal secretory progenitors) (410,411)
|
PDX1
|
GEP EECs (G cells, some intestinal EECs, and pECs
|
Patterning and specification of gastric and duodenal EECs (G cells, SST- and GIP-expressing duodenal EECs) (412,413); Maintenance of beta cell maturity (414,415)
|
ISL1
|
GEP EECs (some gastric EECs**, non EC intestinal EECs, and pECs)
|
Specification of non-EC intestinal EECs (416); maturation and survival of pECs in development (417,418); maintenance of adult beta cell function (419)
|
RFX3
|
pECs
|
pEC differentiation during development (420); differentiation and maintenance of mature beta cells in the adult (421)
|
RFX6
|
GEP EECs (gastric EECs**, some intestinal EECs, and pECs)
|
Differentiation of intestinal EECs (K, L, X, I, and EC cells) (184,422); pEC specification and differentiation in development (423,424); Regulation of insulin expression in beta-cells (425)
|
PAX4
|
GEP EECs (some gastric EECs, duodenal EECs, some pECs)
|
Differentiation of EECs in the duodenum, of EC cells and D cells in the stomach (178), and of beta and delta cells in the pancreas (426,427)
|
PAX6
|
GEP EECs (some gastric EECs, some duodenal EECs, some pECs)
|
Differentiation of D and G cells in the stomach, of K cells in the duodenum (178), and of alpha and epsilon cells in the pancreas (428,429)
|
NKX2.2
|
Some intestinal EECs and some pECs
|
Regulation of cell fate within the intestinal EEC population (promotes EC, L-I-N, and K lineages) (179) and within the pEC population (promotes beta cell, alpha cell, and PP cell lineages) (252)
|
NKX6.1
|
Some gastric EECs and some pECs
|
Differentiation of EC cells in the stomach**(430) and of beta cells in the pancreas (253)
|
NKX6.2
|
Some pECs
|
Differentiation of alpha, beta, and PP cells in the pancreas (431,432)
|
NKX6.3
|
Some gastric EECs
|
Differentiation of G cells in the stomach (433)
|
MAFA
|
Some pECs
|
Maintenance of beta cell identity in the adult pancreas (257,434)
|
MAFB
|
Some pECs
|
Differentiation of alpha and beta cells in the developing pancreas (246,247); Maturation of alpha and beta cells in the adult pancreas (247,256)
|
ARX
|
GEP NE cells (some gastric EECs, some intestinal EECs, and some pECs)
|
Differentiation of G cells in the stomach (435), of L-, I-, and K cells in the intestine (435), and of alpha cells in the developing pancreas (244,245); Maintenance of alpha cell identity in the adult pancreas (436)
|
FOXA2
|
Some intestinal EECs and some pECs
|
Differentiation of L cells and D cells in the intestine (437); Maintenance of adult beta cell maturation in the pancreas (438,439); Maturation of alpha cells in the developing pancreas (440)
|
HHEX
|
Some intestinal EECs and some pECs
|
Differentiation of and maintenance of delta cell identity in the pancreas (262)(implicated in delta cells of the intestine (184,195))
|
* Role in PNECs no well defined
** Role in gastric EECs implicated by expression pattern
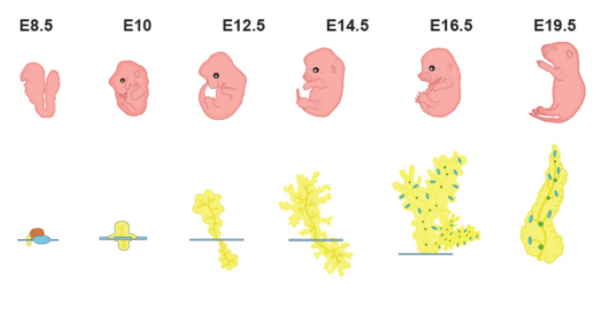
Figure 5. Rodent pancreas development. Schematic depicting the development of the murine pancreas. Islets of Langerhans containing the hormone-secreting endocrine cells are shown in blue and green.
PRIMARY TRANSITION
The first transition phase starts at around embryonic day (E) 9.0-9.5 with the formation of the pancreatic buds and ends at around E12.5 with the start of branching morphogenesis. During this period, the dorsal bud gives rise to the first pancreatic endocrine progenitor cells. As is the case for intestinal EECs, the formation of pECs depends on the expression of the bHLH transcription factor NEUROG3, which is transiently expressed at a relatively low level starting at ~E9.0-E11.0. Mice with targeted disruption of Neurog3 lack pECs and die postnatally of diabetes (232). Consistent with this data in mice, human induced pluripotent stem cells (iPSCs) with null alleles of NEUROG3 fail to differentiate into pECs (233). Following this first pulse of Neurog3 expression, a limited number of pancreatic progenitors becomes committed to the pEC lineage, and some of these cells begin to express glucagon (234).
SECONDARY TRANSITION
Branching morphogenesis continues throughout the first half of the second transition, (~E12.5-E15.5), during which the plexus expands and segregates into tip and trunk domains. From E16.5-E18.5 the epithelial plexus is remodeled into a ductal network with a tree-like structure. Inter- and intralobular ducts branch off from the main pancreatic duct, which connects the pancreas to the common bile duct. During this period, multipotent pancreatic progenitor cells, which make up the majority of cells at the beginning of this transition phase, become either unipotent, fated to become acinar cells at the tip, or bipotent, with the potential to form endocrine and ductal cells. These lineage commitments coincide with a second, more pronounced wave of Neurog3 expression that peaks at around E14.5-E15.5, and then rapidly decreases at around E17.5(235). This second wave of Neurog3 expression commits progenitors to the pEC fate and primes them towards one of the five pEC types.
TERTIARY TRANSITION
During the tertiary transition, individual progenitor cells that have now become fated to the pEC fate by the second, higher pulse of Neurog3 expression delaminate from the ductal network through what is thought to be asymmetric cell division and/or epithelial to mesenchymal transition (EMT). These pECs then migrate throughout the mesenchyme until they encounter other pEC-fated cells, aggregate with the help of Neural cell adhesion molecule (NCAM), and form oval shaped proto-islets (236,237). This process is reminiscent of the way, during lung development, PNECs form NEBs. The formation of fully mature islets requires their vascularization by endothelial cells and their innervation by neurons, both processes that take place from E16.5 onwards, and during the first weeks postnatally.
POSTNATAL
At birth the majority (more than 80%) of the pECs originates from endocrine progenitors and the remainder results from proliferation of preexisting pECs within the islets.
DIFFERENCES IN PANCREATIC ENDOCRINE CELL EVELOPMENT BETWEEN RODENTS AND HUMANS
Although studies of human pEC development are limited, they have uncovered a few main differences compared to rodents. First, there seems to be only one pulse of NEUROG3 during the formation of human pECs (compared to two in the mouse), which occurs at around 8 weeks of gestation. Second, the premature proto-islet structures described above are formed earlier in human development (at 12 weeks post conception) (238,239). Finally, the mature islet architecture differs between rodents and humans (Figure 6). Rodent islets have a central core of beta cells, which are also the most common of all the islet cells (60–80%). The remainder of the endocrine cells, alpha cells (15–20% of the cells of the islet), delta cells (<10% of islet cells), and gamma/ PP cells (<1% of cells) surround the beta cells in a circular structure known as the mantle (240). Human islets do not display the same organized structure as rodent islets. Instead, there is a salt and pepper pattern where the different endocrine cells are randomly scattered within the islets. The proportion of the different pECs also differs as human islets have proportionally less beta but more alpha cells compared to rodent islets, consisting of circa 30% alpha cells, 60% beta cells, about 10% delta cells, and rare <1% gamma/PP cells and epsilon cells (241,242).
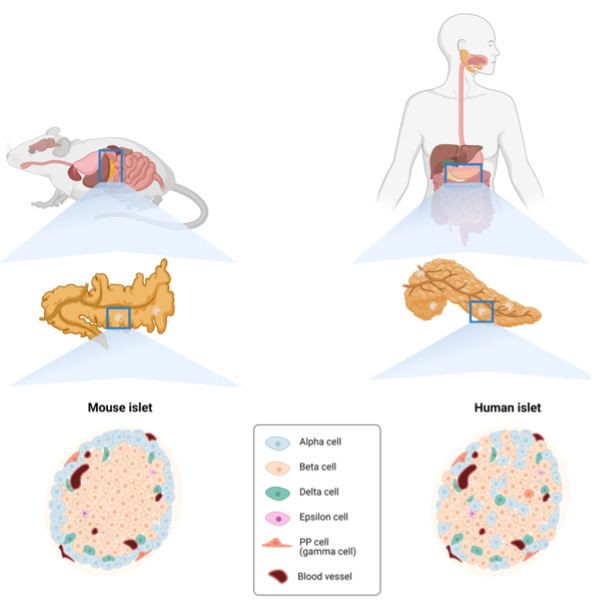
Figure 6. Differences between the murine and human pancreas. Schematic showing the anatomical differences between the murine and human pancreas in terms of both organ and islet architecture.
Pancreatic Islet Cells and Hormones – All with Their Own Function
This section describes the molecular and cellular characteristics of the different subtypes of pECs and their specific hormones and function during adult homeostasis (Figure 7).
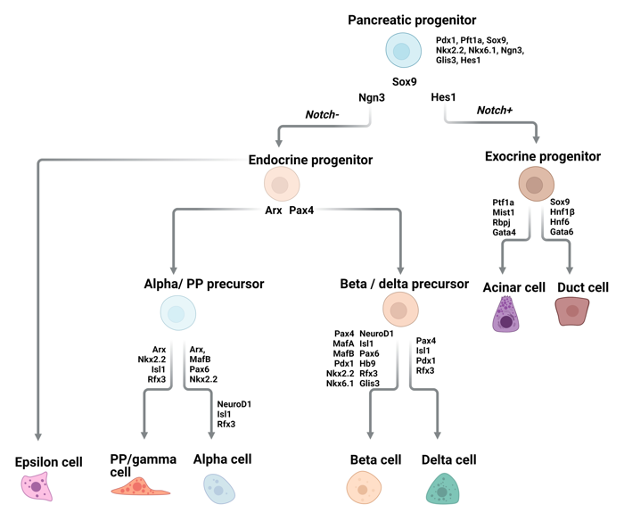
Figure 7. Pancreatic endocrine cells. Diagram of signaling and transcription factor interactions that regulate pancreatic endocrine cell differentiation.
ALPHA CELLS, GLUCAGON
Alpha cells secrete glucagon and are the second most common pEC subtype. Glucagon contributes to maintaining homeostatic blood glucose levels by stimulating glucose production and inhibiting glycogen storage by the liver. The glucagon receptor, GCGR, is widely expressed by multiple organs besides the liver, and glucagon mediates multiple other physiological processes including amino acid metabolism, glomerular filtration by the kidney, lipolysis, and gastric motility (243).
The key factor promoting commitment of pEC progenitors to alpha cells is the transcription factor ARX. Deletion of Arxin Pdx1-expressing progenitors in the mouse leads to a complete loss of alpha cells, accompanied by a compensatory increase in the number of beta and delta cells, which results in the same total numbers of pECs in mutant and wildtype mice (244). Similarly, overexpression of Arx in the embryonic mouse pancreas or in developing islet cells results in an increase in the number of alpha and PP cells while diminishing the number of beta and delta cells (245). While ARX is required for alpha cell specification, the transcription factor, MAFB, is required for their final maturation and hormone expression. Mice with null alleles of MafB show a 50% reduction in insulin and glucagon positive cells (246). Moreover, adult mice in which MafB has been conditionally deleted in either Neurog3-expressing pEC progenitors or in mature pECs had reduced numbers of glucagon-positive cells (247).
BETA CELLS, INSULIN
The most well-known and abundant cell type of the Islets of Langerhans is the beta cell. Beta cells produce insulin which lowers the blood glucose levels via direct and indirect effects on target tissues. Binding of insulin to its receptor facilitates glycolysis in liver hepatocytes and skeletal muscle cells and promotes lipogenesis in the liver and white adipose tissue. Additionally, insulin inhibits hepatic gluconeogenesis and glucagon production by alpha cells (248–251).
Some of the most important transcription factors involved in the differentiation of beta cells are NKX2.2, NKX6.1, NEUROD1, MAFA, and MAFB. In mice lacking the homeodomain transcription factor NKX2.2, beta cell precursors fail to fully mature or produce insulin and, as a consequence, the mutant mice develop hyperglycemia and die shortly after birth (252). NKX2.6 lies downstream of NKX2.2, and in mice lacking Nkx6.1 beta cell neogenesis during the second transition is blocked, and the development of fully differentiated beta cells is prevented (253). NEUROD1 is also required for beta cell maturation (254). Neurod1 null mice have reduced beta cell numbers and fail to develop mature islets (255). Differentiating beta cells express both MafA and MafB, but fully mature beta cells in the mouse only express MafA. Conditional deletion of MafB in the mouse pancreas delayed beta cell maturation (247). Notably, MAFB is expressed in adult human beta cells and while human pluripotent stem cells with MAFB knockout are capable of pEC differentiation, they favor delta cell and PP cell specification at the expense of beta cells (256). MAFA is not essential for beta cell development but is required for insulin secretion and for maintenance of the beta cell identity (257).
DELTA CELLS, SOMATOSTATIN
Pancreatic delta cells, which make up ~5-6% of all islet cells, are responsible for the production of SST. Like insulin and glucagon, SST is a peptide hormone and cleavage of the precursor pro-hormone gives rise to two active isoforms, a long form that acts primarily in the central nervous system and a short form that acts on the organs of the GEP system. Delta cells predominantly secrete the short isoform of SST in response to a variety of stimuli including acetylcholine, glutamate, GLP-1, and urocortin3 produced by beta cells, ghrelin produced by epsilon cells, and high blood glucose levels (258). Within the islets, SST binds to one of its five different receptors on the surface of beta cells and alpha cells, thereby inhibiting the secretion of insulin and glucagon (259,260). SST also acts on cholangiocytes in the liver, inhibiting their secretion of fluids and thereby mediating bile flow (261).
The delta cell-specific transcription factor, haematopoietically expressed homeobox (HHEX), is required for both differentiation of delta cells during embryogenesis and maintenance of their identity in the adult. Deletion of Hhex in mouse pEC progenitors during development led to loss of delta cells by E16.5. Likewise, deletion of Hhex in adult mouse pancreatic delta cells led to a reduced number of delta cells and reduced secretion of SST (262). The phenotype of mice lacking HHEX in the pancreas speaks to the hormonal interplay between pECs: reduced SST secretion in mutant mice led to increased hormone secretion from alpha and beta cells in response to different stimuli. Indeed, despite not being expressed in beta cells, HHEX has been repeatedly identified through GWAS studies as a locus conferring susceptibility to T2D (263).
EPSILON CELLS, GHRELIN
Epsilon cells, described for the first time in 2002, were the last islet cell type to be discovered. The number of pancreatic epsilon cells is highest during embryogenesis, comprising up to 10% of islet cells at mid-gestation, but their numbers then decrease such that they make up only a bit more than 1% of islet cells in the adult pancreas (264). Scattered throughout the islets, epsilon cells produce the growth hormone secretagogue ghrelin. The name of this hormone comes from the Proto-Indo-European root “ghre-”, meaning "to grow," which seems appropriate for a hormone that was discovered in efforts to identify the ligand for the growth hormone secretagogue receptor (GHS-R) (265). Also known as the hunger hormone, ghrelin is a 28 amino acid peptide that needs to be post-translationally acetylated before it can bind to its receptor, GHS-R, and exert its function on its target cells in an expanding list of target organs (266).
Consistent with its stimulatory effect on food intake, ghrelin concentrations are highest during fasting. This function is primarily mediated by ghrelin secreted by gastric X cells. The other hallmark functions of ghrelin are stimulating fat deposition and stimulating growth hormone release from the pituitary. The list of functions attributed to ghrelin is still growing and includes regulating glucose and energy homeostasis, cardioprotection, muscle atrophy, and bone metabolism (267). Secretion of ghrelin is stimulated by glucagon and is inhibited by glucose, insulin, leptin, and GLP-2(268–270). In the pancreas, ghrelin secreted by epsilon cells binds to the GHS-R on delta cells, thereby inducing them to secrete SST, which in turn inhibits the secretion of insulin by beta cells (271,272). There is also some evidence that ghrelin promotes the survival and proliferation of beta cells (264).
A complex interaction between NKX2.2 and NEUROD1 appears to be involved in epsilon cell specification during development (264). However, the transcription factor most closely linked specifically to epsilon cells is PAX6, which appears to inhibit epsilon cell formation. Ghrelin-expressing cells are increased in the developing pancreas of Pax6 knock-out mice at the cost of alpha cells (273). Likewise, when Pax6 was deleted in the adult pancreas of mice, the same increase in epsilon cell numbers was observed, concomitant with loss of beta cells, alpha cells, and delta cells (274). A later study demonstrated that, upon loss of Pax6 expression, beta and alpha cells began to express ghrelin (275).
PP (GAMMA) CELLS, PANCREATIC POLYPEPTIDE
PP cells comprise less than 1-2% of the islets and the majority are located in the head of the pancreas. Perhaps due to their scarcity in the islets, not much is known about the genetic determinants of PP cell specification and maturation. Signals from the vagus nerve, enteric neurons, and arginine following meal intake stimulate PP cells to release pancreatic polypeptide (PP) (276). This hormone has the opposite effect of ghrelin and is referred to as the satiety hormone (277). Transgenic mice that overexpress PP show reduced weight gain and decreased fat mass, and long-acting PP analogues are being explored for the treatment of human obesity (278,279). Within the pancreatic islet, PP indirectly affects insulin secretion by inhibiting glucagon and SST secretion via a receptor expressed on alpha and delta cells, respectively (280,281).
Other members belonging to the same peptide hormone family as PP are neuropeptide Y (NPY) and peptide YY (PYY) which are produced in the GI tract. PYY is produced by L cells in the intestine and colon and its function mimics that of PP in the pancreas (282).
Pancreatic Endocrine Cells in Injury Repair
Injury to the pancreatic epithelium in the form of surgery, inflammation or metabolic trauma disrupts homeostasis by causing extensive cell loss. Failure in injury repair can result in organ dysfunction and cause clinical symptoms.
The youthful pancreas has a high potential to regenerate damaged tissue following injury (283,284). In contrast to the GI tract, however, where this capacity to regenerate is maintained throughout life, the regenerative capacity of pECs is limited in adult tissue (285,286). Through observations made in rodent injury models, mostly looking at beta cells, it has become clear that the endocrine pancreas employs multiple strategies to regain homeostasis following injury.
REPLICATION OF PRE-EXISTING BETA CELLS
The ability of pre-existing beta cells to replicate in response to injury was first shown by the Melton lab. Using a beta-cell-specific lineage trace they found that the newly generated beta cells that arose following partial pancreatectomy originated from pre-existing ones (287). Using the same injury model, but an unbiased thymidine analogue based labelling technique, a different group came to the same conclusion (288).
NEOGENESIS OF BETA CELLS FROM A STEM/PROGENITOR CELL
An alternative hypothesis is that beta cell regeneration in the adult pancreas involves a process called neogenesis, which involves the formation of de novo beta cells derived from a stem or progenitor cell expressing Neurog3. Initial studies of neogenesis were based on in vivo pulse labelling with the thymidine analogue, BrdU. In one study, IFN-gamma induced beta cell depletion resulted in the appearance of budding duct/islet-like structures containing proliferative ductal cells as well as newly formed acinar and endocrine cells (289). Similar observations were made in two different models of pancreatic injury in rats, where regeneration involved marked proliferation of ductal cells, some of which expressed ductal and endocrine markers, followed by the formation of new pECs and islet structures (290,291). Researchers from the Heimberg lab found that, subsequent to pancreatitis induced by pancreatic duct ligation in mice, the region of the pancreas undergoing regeneration contained NEUROG3-positive cells, a portion of which also expressed ductal markers (292). Finally, a lineage trace of ductal cells in mice showed that, following injury, ductal, acinar, and endocrine cells all contained the lineage trace (293). Altogether, these studies suggest that, following injury, new pECs can be derived from a progenitor cell in the pancreas ductal epithelium, whose differentiation to the pEC fate is dependent on transient expression of Neurog3.
TRANS-DIFFERENTIATION OF NON-BETA CELLS INTO BETA CELLS
Finally, a landmark study from the lab of Pedro L. Herrera, provided evidence for yet another strategy employed by the pancreatic endocrine compartment to regenerate. Prior to inducing genetic ablation of adult beta cells, the authors induced a lineage trace of glucagon-producing alpha cells. In the initial months following beta cell ablation, mice required supplementation with insulin. However, at 6 months post-ablation, mice no longer required supplemental insulin and their pancreata showed increased beta cell mass and beta cells that expressed both insulin and glucagon. At early time points following ablation, the majority of the regenerated beta cells in these mice contained the alpha cell lineage trace, arguing that the beta cells had resulted from trans-differentiation of the alpha cells (294). This provided new evidence of endocrine cell plasticity.
Pancreatic Endocrine Cell Hyperplasia
In the previous section we discussed observations relating to pEC regeneration in the context of conditions that lead to direct loss of pECs. Given defining pEC characteristics such as direct innervation and the role of pECs in mediating a number of physiological processes, it is likely that pECs also respond to stimuli produced in the context of other pathological conditions. As we have discussed in previous sections of this text, EEC and PNEC hyperplasia have each been observed in the context of inflammation or injury of their respective tissue sites. Likewise, an increase in the number pECs has been observed as a response to some pathological conditions relating to the pancreas (295,296). Moreover, focal endocrine hyperplasias have been observed incidentally in the pancreas of up to 10% of screened adults at autopsy (297).
The pEC mass is normally 1% and 3% of the total pancreatic mass in adults and infants, respectively. If this increases to more than 2% or 10% of the total pancreatic mass in adults or infants, respectively, it is defined as pEC hyperplasia (222,297,298). Some instances of pEC hyperplasia involve a general increase in the size of pancreatic islets that results in an increase in overall islet cell numbers but not in a change in the relative frequencies of one pEC subtype versus another. However, the majority of pEC hyperplasia are associated with an increase in the number of a specific subtype of pEC, most commonly alpha and beta cells. Hyperplasia of other pEC subtypes including the rare gamma/PP cells have also been reported. Morphologically, pEC hyperplasia either appears as large islets (larger than 250 mm in diameter) or as budding structures protruding from the ductal epithelium (297). The latter budding structures are reminiscent of the structures described above in mouse models of pEC injury and are suggestive of pEC neogenesis.
BETA CELL HYPERPLASIA
Beta cell hyperplasia is commonly observed in patients with insulin resistance and early T2D and is likely a physiological response to these conditions. Other clinical conditions in which beta cell hyperplasia is implicated include persistent hyperinsulinemic hypoglycemia of infancy (PHHI) and non-insulinoma pancreatogenous hypoglycemia syndrome (NIPHS) (299–302). These conditions are associated with dysregulated insulin secretion and hypoglycemia in infants and neonates or in adults, respectively.
The pancreas of patients with PHHI is characterized by the presence of either focal beta cell hyperplasia, resulting in a focal increase in islet size or, more commonly, of diffuse beta cell hyperplasia in which enlarged islets and small, irregularly shaped endocrine cell clusters are found throughout the pancreas (297). The percentage of beta cells present within the islets of patients with PHHI is increased such that they account for 70-90% of the islet (222). Increased proliferation of not just beta cells but also of ductal and centroacinar cells has been reported in the pancreas of patients with PHHI (303). PHHI is caused by mutations in ABCC8 and KCNJ11, which encode for subunits of the ATP-sensitive potassium channel involved in insulin secretion, as well as by mutations in genes affecting beta cell metabolism such as glucokinase (GCK), glutamate dehydrogenase (GLUD1), and short chain fatty acid hyroxyacyl dehydrogenase (SCHAD) (304).
NIPH is defined by postprandial hypoglycemia and, unlike PHHI, the genetic cause has not been clearly identified. The pancreas of patients with NIPH exhibits an increase in both number and size of the islets, and contains endocrine cells budding from the ductal epithelium (305,306). Symptoms of both PHHI and NIPH can be resolved through either partial or near total pancreatectomy.
ALPHA CELL HYPERPLASIA
Alpha cell hyperplasia (ACH) is a rare condition most commonly caused by mutations in the gene that encodes for the glucagon receptor, GCGR (307,308). The number of islets in patients with ACH is increased and the islets vary in size, are often larger, and contain a high proportion of alpha cells (309). Patients with ACH often, though not always, also present with hyperglucagonemia, and multiple pancreatic NETs. The multifocality of ACH and pancreatic NET lesions in these patients, and the observed presence of large islets showing signs of morphological transition from ACH to glucagonomas suggests that ACH lesions can progress to frank glucagonomas (308,309). Interestingly, these patients do not display features of glucagonoma syndrome due to their GCGR mutations. Mice with germline null mutations in Gcgr, or with liver-specific deletion of Gcgr also develop ACH that can progress to glucagonomas. Pharmacologic interruption of glucagon signaling in mice also leads to ACH. In these models, ACH appears to be primarily driven by alpha cell proliferation, though it is possible that transdifferentiation of other pECs or of ductal cells is also involved. It is interesting to note that the clear link between GCGR function and ACH implies a signaling feedback loop that regulates both the number of glucagon-producing alpha cells and their secretion of glucagon. One of the signals that is likely to contribute to ACH is amino acids. Serum amino acid levels are increased in patients with ACH and transcriptomic analysis of mouse models of ACH have shown altered expression patterns for genes involved in amino acid catabolism and transport (310).
GAMMA/PPCELL HYPERPLASIA
Compared to alpha and beta cell hyperplasia even less is known about PP cell hyperplasia, which occurs very rarely. As with alpha and beta cell hyperplasia the number and size of the pancreatic islets in patients with PP cell hyperplasia is increased and contain a high proportion of PP cells (311–313). In 50% of the reported PP hyperplasia cases, the patients had suffered from gastrinoma or ZES. In addition, there is no correlation between PP cell number and PP serum levels. It is possible that PP hyperplasia arises as an effect of gastrinomas (297). There are also no genetic changes directly related to the onset of this condition.
GEP NEUROENDOCRINE NEOPLASMS (GEP-NENs)
GEP-NENs encompass all NENs that arise along the GEP tract and account for 55 to 70% of NENs from all tissue sites (82,314,315). GEP-NENs comprise both well-differentiated NETs and poorly differentiated NECs. In addition, some GEP-NENs occur as GEP-mixed neuroendocrine/non-neuroendocrine neoplasms (GEP-MiNEN) that can be either well- or poorly- differentiated and are characterized by their mixed morphology showing endocrine and non-endocrine features. Based on proliferation (measured by mitotic count and Ki67 index) GEP-NETs are further subdivided into low (G1), intermediate (G2), and high (G3) grade NETs (82). G3 well-differentiated NETs are associated with a poor prognosis and show a decidedly more aggressive clinical behavior than G1 and G2 GEP-NETs. G3 well-differentiated NETs are more commonly observed in the pancreas than in other GEP tissue sites. GEP-NECs can be subdivided into small cell NEC and large cell NECs.
GEP-NECs
GEP-NECs comprise 10-20% of all NENs, with roughly 38% arising in the GI tract (colon, anus, rectum) and 23% in the pancreas (316). GEP-NETs and GEP-NECs display different mutational profiles and are therefore considered separate disease entities. Indeed, the most common site for NECs is the large bowel, whereas the most common site for NETs is the ileum (316). In addition, the observations that some GI-NECs show features resembling adenocarcinomas or squamous cell carcinomas and that up to 40% of NECs show non-endocrine features, have led some researchers to hypothesize that GEP-NECs are more closely related to non-endocrine tumor types than to high grade (G3) NETs (82,317,318).
The importance of the two genes RB1 and TP53 in the genesis of NECs is highlighted by the fact that these genes are commonly mutated in both lung-NECs and GEP-NECs. TP53 mutations have been identified in 20-73% of all GEP-NECs (319–323) while RB1 mutations have been identified in 44-86% of all GEP-NECs (322,324–326). The limited number of studies that have performed genomic analyses of GEP-NECs have also identified other genes that are commonly mutated in GEP-NECs, including KRAS, SMAD4 and APC (318,327,328). In addition, GEP-NECs are characterized by frequent and severe chromosomal abnormalities (79).
As discussed previously, generating a GEMM of SCLC was achieved through conditional tissue-specific deletion of Rb1 and p53 in the mouse lung epithelium (89). In contrast, attempts to generate GEMMs of RB1; TP53 mutant GEP-NECs by deletion of Rb1 and p53 in targeted mouse GEP tissues have proven to be less straightforward and have given mixed results (329). Conditional deletion of Rb1 and p53 in renin-expressing mouse pECs led to the generation of highly aggressive, metastatic, glucagon-producing tumors (330). Given the expression of glucagon in combination with the aggressive course of the tumors developed by this GEMM, however, it is unclear whether they are a more suitable model for sporadic glucagonomas or for pancreatic NECs. In human patients, pancreatic NECs are almost exclusively non-functional, i.e., they do not secrete symptom-inducing hormones (331). In the RIP-Tag2 GEMM, instead, SV40 T-antigen is expressed in beta cells, thereby effectively abrogating the Rb1 and p53 pathways in these pECs. RIP-Tag2 mice primarily develop aggressive insulinomas and, to a lesser extent, poorly differentiated pancreatic NECs (332). The RIP-Tag2 model has been instrumental in delineating stepwise aspects of pancreatic NEN tumorigenesis and in identifying therapeutic strategies for these tumors in patients (329). Nonetheless, whether this GEMM can be used as a reliable model for pancreatic NECs is unclear.
In addition to GEMMs, cell lines and, more recently, GEP-NEC patient-derived tumor organoid lines have been generated (333,334). Patient-derived tumor organoids, 3D long-term cultures of tumor cells, can be expanded long-term, can be cryopreserved, and have been shown to be representative of the patient tumor tissue from which they were derived at both the genetic and phenotypic levels (335). Recently, a genetically engineered organoid model of GEP-NECs was generated by CRISPR/Cas9 mediated compound knock-out of RB1 and TP53 combined with overexpression of 6 transcription factors in otherwise normal colon organoids (333). Interestingly, in the absence of transcription factor overexpression, compound knock-out of RB1 and TP53 was not sufficient to generate GEP-NECs from these cells.
Together with genomic analyses of GEP-NECs, studies using preclinical models of GEP-NECs have been informative. Nonetheless, to date, strategies for stratifying patients in terms of the molecular characteristics of their tumors and the likely response of their tumors to specific therapies are lacking. Currently, the primary treatment strategies for GEP-NECs use platinum agents combined with etoposide, based on the relative effectiveness of this approach in treating SCLC, which is a more common and better studied NEC subtype (336–338). Overall, the response rate for GEP-NECs to first line therapy is 40-60%. Upon relapse or the tumors becoming refractory, there are no well-established second-line therapies. This is reflected in a median survival of 38 months in patients with localized disease and only 5 months in patients with metastatic disease (339). Other potential treatment strategies for patients with GEP-NECs that are currently undergoing clinical trials are the mTOR inhibitor everolimus and some forms of immunotherapy (336–338).
GEP-NETs
GEP-NETs represent 80-90% of all GEP-NENs and comprise many different tumor types. GEP-NETs are classified as functional or nonfunctional depending on whether they secrete symptom-causing hormone peptides. Functional GEP-NETs, which can arise throughout the GEP-tract, include gastrinomas and insulinomas (10). GEP-NETs are highly heterogeneous with regards to their biological behavior and clinical presentation, course, and prognosis. The most common tissue sites of primary GEP-NETs are the small intestine, the rectum, the colon, the pancreas (12.1%), and the appendix (315). In general, G1 and G2 GEP-NETs are associated with high 5-year survival rates ranging from 75 to 79% and from 62 to 74%, respectively. The 5-year survival rate for patients with G3 well-differentiation NETs, on the other hand, shows more variability between NETs of the intestine (40%) and NETs of the pancreas (7%) (340). Notably, the recognition of the category of G3 well-differentiated pancreatic NET by the WHO in 2017 has had important implications for patients and clinicians, as it highlighted the fact that some pancreatic NETs, despite showing morphological features and differentiation more commonly associated with low-grade NETs, show aggressive behavior more similar to that of NECs (82,331).
The most prevalent sites of origin for GEP-NETs show regional differences that are likely reflective of differences in both environmental and genetic factors. GI-NETs arising from the small intestine or colon are most common in the USA, small intestinal or pancreatic NETs are more common in Europe, while in Asia gastric and rectal NETs are most prevalent (341). The most well studied GEP-NETs are pancreatic NETs and SI-NETs.
Gastric NETs (G-NETs) are relatively rare and only account for 4 to 6% of NENs (342,343). These tumors are classified into one of four categories according to their clinical characteristics. Most G-NETs are type I tumors, which are associated with CAG and arise as multiple small nodules. These tumors rarely metastasize. Type II G-NETs are very similar to type I tumors but are commonly associated with MEN1 syndrome (in conjunction with gastrin producing pancreatic NETs) or ZES. Type I and type II G-NETs arise as a consequence of excessive gastrin. Type III G-NETs are sporadic tumors that are not associated with other gastric conditions and present as large, solitary lesions. Finally, type IV tumors, G-NECs, are both the very rare and the most malignant. These tumors arise sporadically and are poorly differentiated. While type I, II, and III G-NETs are ECL cell tumors, type IV G-NECs arise from other endocrine cell types (344).
Small intestinal NETs (SI-NETs) are the most common neoplasm arising in the small intestine (345). They include NETs arising in the jejunum and ileum, with the ileum being the major site of incidence (346). A unique feature of ileal SI-NETs is that they are multifocal in 10-20% of cases, with the different tumors arising independently (347). Moreover, ileal SI-NETs have a high rate of metastasis, with >50% of ileal SI-NET patients presenting with metastases at the time of diagnosis (348). Finally, the majority of SI-NETs produce serotonin, causing many of these to induce carcinoid syndrome, the most common functional hormonal syndrome of patients with NETs (349). Clinical symptoms of carcinoid syndrome include watery diarrhea, flushing, hypotension, breathlessness, wheezing, and loss of appetite, all attributable to not just increased serum levels of serotonin but also of prostaglandins, histamine, bradykinin, and tachykinins (9,350). Carcinoid syndrome is most often observed when NETs, having metastasized to the liver, secrete their biologically active compounds directly into the systemic circulation (351). Carcinoid syndrome can cause carcinoid heart disease in which elevated serum serotonin levels cause fibrosis in the heart. Symptoms of carcinoid syndrome attributable to serotonin can be ameliorated through the administration of serotonin-inhibiting therapies (340,352).
Of note, duodenal NETs are sometimes considered separately from SI-NETs as they more closely resemble gastric and pancreatic NETs in terms of their mutational profile (353). Whereas the serotonin producing EC cells are thought to be the cell of origin of SI-NETS, duodenal NETs more commonly express gastrin and somatostatin (described in more detail later in this chapter) and are therefore thought to arise from G and D cells (354,355).
Pancreatic NETs (PanNETs) are the best studied subtype of GEP-NET and are the only subtype for which the category of high grade G3 well-differentiated NET has been officially recognized (356). While some well-differentiated PanNETs are functional and consist of a single hormone-producing cell type, the majority of PanNETs are non-functional and contain a mixture of cells expressing markers for the different pEC types (357,358). PanNETs are thought to arise from differentiated endocrine cells of the islets of Langerhans. However, a recent study based on next generation DNA methylation analysis suggests that they may also arise from the exocrine pancreas (359).
Familial GEP-NETS
While the majority of the GEP-NETs arise sporadically, approximately 5% occur as part of a hereditary cancer predisposition syndrome (360). The most common of these syndromes are associated with the development of duodenal and PanNETs and are caused by germline mutation in one of five different genes: MEN1, tuberous sclerosis complex 1 (TSC1), tuberous sclerosis complex 2 (TSC2), Von Hippel–Lindau (VHL), and neurofibromatosis type 1 (NF1) (361). Of these, MEN1 is the gene most strongly implicated in PanNETs. MEN1 encodes for the protein menin, which acts as a scaffold for both transcription factors and chromatin-modifying enzymes (362–364). Thus, although its exact function is yet to be determined, menin has been suggested to play a role in multiple processes including DNA damage repair, cell cycle regulation, histone methylation, and mTOR pathway activity (365).
Familial SI-NETs are far rarer and have been associated with germline mutations in CDKN1B, inositol polyphosphate multikinase (IPMK), and MutY DNA glycosylase gene (MUTYH) (366–369). Notably, germline mutations in CDKN1B have been shown to be causative of the MEN4 familial cancer syndrome in which patients develop parathyroid, pituitary, and, more rarely, SI-NETs (323). Finally, there is one example of a single consanguineous family in which several family members were affected by hypergastrinemia and consequent gastric ECL NETs. These familial G-NETs were shown to be caused by germline inactivating mutations in the gene for a proton pump expressed by parietal cells and involved in gastric secretion, ATP4a (370).
Genetics of Sporadic GEP-NETs
Although rare, familial cancer syndromes associated with NETs have pointed to genes and pathways that are also important for the genesis of sporadic NETs. Genetic studies have revealed that somatic inactivation by mutation, chromosomal alteration, or epigenetic silencing of genes such as MEN1, TSC2, and VHL, each associated with a familial NET syndrome, are found in approximately 40%, 35%, and 25% of sporadic pancreatic NETs (371,372). Familial SI-NET syndromes are rarer, and of the causative genes for these syndromes, only mutations in CDKN1Bhave also been identified in 9% of sporadic SI-NETs (373). Of note, the largest whole genome analysis of pancreatic NETs to date, uncovered germline mutations in CDKN1B and MUTYH in pancreatic NETs from patients that had no family history of the disease, therefore implicating alterations in these genes also in the genesis of pancreatic NETs and, perhaps, of GEP-NETs in general (371,372). Incidentally, germline variants in pancreatic NET samples in this cohort were also identified in checkpoint kinase 2 (CHEK2), and BRCA2 (371,372).
Molecular analysis of pancreatic NETs also uncovered alterations in genes that are not implicated in familial NET syndromes. In particular, Jiao et al. identified recurrent mutations in PTEN and PIK3CA in pancreatic NETs. This study also identified recurrent mutations in the chromatin remodeling enzymes DAXX (death domain associated protein) and ATRX (α thalassemia/mental retardation syndrome X-linked), in 25% and 18% of pancreatic NETs (374). DAXX and ATRX function together in a complex that deposits histone H3.3 at different sites including telomeres and mutations in ATRX/DAXX were mutually exclusive in pancreatic NETs (372). ATRX/DAXX mutations in pancreatic NETs were correlated with chromosomal instability and the activation of a telomerase independent telomere maintenance mechanism, alternative lengthening of telomeres (ALT) (375,376). Perhaps it is thus not entirely surprising that ATRX/DAXX mutations are more often found in G3 pancreatic NETs than G1/G2 pancreatic NETs and are associated with reduced patient survival (323,375). Other mutations found in G3 pancreatic NETs and associated with shorter survival times are mutations in ARID1A and CDKN2a (377,378).
Whereas several driver mutations have been identified in pancreatic NETs, identifying a clear genetic driver of SI-NETs has been more difficult. Instead, the development of sporadic SI-NETs seems to be dependent on chromosomal aberrations. Loss of chromosome (chr) 18 is observed in more than 60% of SI-NETs. While the functional importance of this chromosomal loss in SI-NETs has not been determined, one study identified allelic loss of BCL2, CDH19, DCC, and SMAD4 (all on chr 18) in 44% of SI-NETs (379). Loss of chr 9 and 16 or gain of chr 4, 5, 7, 14 or 20 are also recurrently observed in SI-NETs, albeit at lower frequencies (353,380–383). Targeted mutational and copy number analysis of metastatic SI-NETs has identified recurrent mutations in APC, CDKN2C, BRAF, KRAS, PIK3CA, and TP53, though at relatively low frequencies (ranging from 4 to 10%) (328,379,384).
Although genomic studies demonstrate that SI-NETs and pancreatic NETs have distinct genomic profiles, a common pathway alteration stands out -- activation of mTOR/PI3K signaling. In pancreatic NETs the pathway is recurrently activated by frequent loss of negative regulators of the pathway (PTEN, TSC1/2) and by recurrent activating mutations in PIK3CA. Likewise, this pathway is implicated in SI-NETs by the observation of recurrent activating mutations in KRAS, BRAF, and PIK3CA as well as, more commonly, of frequent copy number gains in components of this pathway, including SRC and mTOR itself (323). Frequent alteration of CDKN family genes also appears to be a common feature of both GEP-NET subtypes, suggesting deregulation of the cell cycle is also important for NETs. Finally, though not discussed in this text, aberrant methylation patterns are likely to contribute to GEP-NETs in general and some studies have suggested that methylation patterns can be used to stratify GEP-NETs with implications for patient prognosis (385–387).
Treatments for GEP-NETs
The main alternatives used to treat GEP-NETs for which surgical resection is not possible are hormone analogs, PRRT, the mTOR inhibitor everolimus, and for pancreatic NETs, sunitinib. Chemotherapy mainly targets proliferative cells and is therefore predominantly used on NECs and G2 pancreatic NETs.
Somatostatin analogues (SSAs) such as octreotide and lanreotide bind to the somatostatin receptors (SSTR1-5) and mimic the natural hormone’s ability to exert an inhibitory function on the cell’s hormone secretion. To prolong their effect, these analogues have been synthetically engineered to have an increased half-life compared to the endogenous hormone. Administration of SSAs is common in the treatment of functional GEP-NETs as they prevent the tumors from secreting excessive amounts of hormones and thus alleviate clinical symptoms (388,389). Although GEP-NETs can express all five somatostatin receptors, the majority express SSTR2. Binding of SSAs to SSTR2 has been shown to decrease proliferation and lead to disease stabilization in GEP-NET patients (390–392). In addition, SSAs can also be coupled to radioactive isotopes and used for targeted radiological therapy. The internalization of the radioactive isotope by the cancer cells causes DNA damage and cell death (393,394).
As discussed previously, the mTOR-AKT pathway plays an important role in GEP-NETs. Consistent with this, inhibitors of this pathway such as everolimus have been shown to have a positive effect (132,395,396). Cell type-specific drugs have also been used in the treatment of pancreatic NETs and one of the oldest drugs is streptozotocin, a beta cell specific cytotoxic agent that has been used for almost four decades (397). Streptozotocin selectively enters beta cells via the glucose transporter, GLUT2, causing DNA damage resulting in cell death (398). Finally, inhibitors of receptor tyrosine kinases, vascular endothelial growth factor and its receptor have been effective in the treatment of GEP-NETs, most notably, pancreatic NETs as they are often highly vascularized (399,400).
CONCLUSIONS
When comparing neuroendocrine cells from different tissues, multiple recurrent themes can be identified. For one, their development relies on the expression of bHLH transcription factors ASCL1 and NEUROG3. Animal models have highlighted the differential roles played by these two transcription factors in the formation and differentiation of NE cells of the diffuse NE system. Whereas ASCL1 drives PNEC lineage commitment in the lung, NEUROG3 is essential for the formation of the intestinal EECs and pECs. Both ASCL1 and NEUROG3 appear to be important for the development of gastric EECs. Another interesting difference can be seen in the expression pattern of these transcription factors. Whereas NEUROG3 is transiently expressed during the formation of GEP endocrine cells, ASCL1 is constitutively expressed in lung progenitor and mature PNECs. The mechanistic differences and biological reason behind these differential dependencies on either ASCL1 or NEUROG3 remain to be determined.
Second, NE cells of the diffuse NE system appear to be involved in the response to some forms of injury, as indicated by their increased numbers in some disease conditions. Importantly, comparing NE cells during development or homeostasis to NE cells in disease conditions has the potential to uncover aberrations in common pathways and regulatory factors that contribute to or mediate the pathological state. This kind of analysis is likely to provide candidate targets for the development of new (targeted) therapies.
Another recurrent theme in the biology of neuroendocrine cells from different tissues, is the crosstalk between NE cells and their environment. This crosstalk can be seen both in the response of NE cells to environmental stimuli and in the direct influence they can exert both locally and systemically through the bioactive compounds they secrete. Finally, the ideas of NE cell plasticity and NE cell heterogeneity are ones that have not been fully explored but might be important to their function. As an example of the former, although EECs are named after their predominantly expressed hormone, they can also be multi-hormonal and even change their hormone expression depending on their location within the tissue. While EEC hormonal switching is just starting to be discovered within the intestinal tract, it is likely that a similar degree of plasticity in hormone expression exists for other NE cell types. Indeed, some hints of plasticity between endocrine cells can also be seen in pancreatic endocrine cells, where some studies imply trans-differentiation of alpha cells to beta cells under certain conditions. Regarding NE cell heterogeneity, it is interesting to note that, whereas the initial studies of NE cells were driven by observations about their shared features, with the advancement of single-cell sequencing technologies, the more recent era of NE cell research has highlighted a previously underappreciated heterogeneity of different tissue-specific NE cell populations (34,35,164,183,401,402). NE cell heterogeneity highlights the plasticity and dynamic nature of these cells as they respond to external stimuli and microenvironmental signals in a context-specific manner.
With regards to NENs, next generation sequencing efforts have started to characterize the mutational landscape of NENs. So far, these have been mostly focused on pancreatic and lung NENs. Similar studies of NENs originating from other organs would be of value as they could provide new insights into NEN biology. Importantly, candidates discovered in genome-wide genomic studies need to be validated to determine whether they represent drivers or passengers throughout disease progression. Additionally, their exact mechanistic function is of importance if they are to become targets for drug development. For NENs with a lower mutational burden and few recurrent mutations, epigenome, non-coding or protein level changes may play a more significant role in disease initiation and progression. These possibilities are just starting to be explored.
A significant challenge in studying NENs is the development of model systems that can be used to study both basic as well as translational NEN biology. Currently, the most commonly used models include GEMMs, cell lines, and patient derived xenografts (PDX). Although these model systems have provided invaluable knowledge about NEN biology they also have their limitations. GEMMs have been instrumental in the study of SCLC and some very specific subtypes of pancreatic NETs (RIP-Tag2 mice and Men1 mutant mice). Nonetheless, the current GEMMs for different NENs do not cover the entire range of different tumor types encompassed by NENs. Furthermore, species differences require comparisons and/or end stage studies in human derived model systems.
Cell lines, which while being robust and relatively cheap to culture, suffer from genetic changes occurring over time and may not recapitulate the full tumor heterogeneity.
PDX models circumvent those limitations while providing analysis in an in vivo environment, but have a very low engraftment rate of <10% and tedious logistics (403). Organoids derived from healthy tissue provide researchers with the means to study normal NE cell differentiation via the establishment of differentiation protocols (183,195) and offer the potential to model NEN disease progression by stepwise genome engineering, as has been achieved for other tumor types (333,404–406). Moreover, in vitro drug screens on patient-derived tumor organoids have the potential to aid personalized treatment. However, to date only a handful of NEN organoid lines have been established. Of note, most of those organoid lines represent NECs, rather than NETs (333,334).
Although NEN biology is starting to be explored in more detail, much remains to be discovered. A combination of both basic and translational research will be needed to provide the biological insights necessary to significantly be able to improve or establish novel clinical treatment options for NENs.
ACKNOWLEDGEMENTS
We thank Lisanne den Hartigh for help with making figure 5 and Joep Beumer for helpful discussions. Figures 1 - 4 and 6-7 were created with BioRender.com. This work was supported by an EMBO long-term fellowship (AALTF 332-2018 to A.A.R.) and funding from the Neuroendocrine Tumor Research Foundation under a Petersen Accelerator Award (H.C. and T.D.).
REFERENCES
- Tischler AS. The dispersed neuroendocrine cells: the structure, function, regulation and effects of xenobiotics on this system. Toxicol. Pathol. 1989;17(2):307–316.
- Røseth A, Chapman R, Ramachandran R, Sheehan C. Chapter 9.16 - Gastrointestinal Tract. In: Wild D, ed. The Immunoassay Handbook (Fourth Edition). Oxford: Elsevier; 2013:891–900.
- Rosai J. The origin of neuroendocrine tumors and the neural crest saga. Mod. Pathol. 2011;24 Suppl 2:S53–7.
- Rindi G, Leiter AB, Kopin AS, Bordi C, Solcia E. The “normal” endocrine cell of the gut: changing concepts and new evidences. Ann. N. Y. Acad. Sci. 2004;1014:1–12.
- Lechago J. The endocrine cells of the digestive tract. General concepts and historic perspective. Am. J. Surg. Pathol. 1987;11 Suppl 1:63–70.
- Modlin IM, Champaneria MC, Bornschein J, Kidd M. Evolution of the diffuse neuroendocrine system--clear cells and cloudy origins. Neuroendocrinology 2006;84(2):69–82.
- de Herder WW, Rehfeld JF, Kidd M, Modlin IM. A short history of neuroendocrine tumours and their peptide hormones. Best Pract. Res. Clin. Endocrinol. Metab. 2016;30(1):3–17.
- Day R, Salzet M. The neuroendocrine phenotype, cellular plasticity, and the search for genetic switches: Redefi ning the diffuse neuroendocrine system. Oct-Dec 2002;2.
- Champaneria MC, Modlin IM, Kidd M, Eick GN. Friedrich Feyrter: a precise intellect in a diffuse system. Neuroendocrinology 2006;83(5-6):394–404.
- Hofland J, Kaltsas G, de Herder WW. Advances in the Diagnosis and Management of Well-Differentiated Neuroendocrine Neoplasms. Endocr. Rev. 2020;41(2). doi:10.1210/endrev/bnz004.
- Linnoila RI. Functional facets of the pulmonary neuroendocrine system. Lab. Invest. 2006;86(5):425–444.
- Frohlich F. The Light Cell Of the Bronchial Mucosa and Its Relationship to the Problem Of Chemoreceptors. Frankf. Z. Pathol. 1949;60(3-4):517–59, illust.
- Bensch KG, Corrin B, Pariente R, Spencer H. Oat-cell carcinoma of the lung. Its origin and relationship to bronchial carcinoid. Cancer 1968;22(6):1163–1172.
- Lauweryns JM, Cokelaere J, Theunynck P. Serotonin producing neuroepithelial bodies in rabbit respiratory mucosa. Science 1973;180(4084):410–413.
- Garg A, Sui P, Verheyden JM, Young LR, Sun X. Consider the lung as a sensory organ: A tip from pulmonary neuroendocrine cells. Curr. Top. Dev. Biol. 2019;132:67–89.
- Lauweryns JM, Peuskens JC. Neuro-epithelial bodies (neuroreceptor or secretory organs?) in human infant bronchial and bronchiolar epithelium. Anat. Rec. 1972;172(3):471–481.
- Van Lommel A, Bollé T, Fannes W, Lauweryns JM. The pulmonary neuroendocrine system: the past decade. Arch. Histol. Cytol. 1999;62(1):1–16.
- Noguchi M, Furukawa KT, Morimoto M. Pulmonary neuroendocrine cells: physiology, tissue homeostasis and disease. Dis. Model. Mech. 2020;13(12). doi:10.1242/dmm.046920.
- Xu J, Yu H, Sun X. Less Is More: Rare Pulmonary Neuroendocrine Cells Function as Critical Sensors in Lung. Dev. Cell 2020;55(2):123–132.
- Van Lommel A. Pulmonary neuroendocrine cells (PNEC) and neuroepithelial bodies (NEB): chemoreceptors and regulators of lung development. Paediatr. Respir. Rev. 2001;2(2):171–176.
- Branchfield K, Nantie L, Verheyden JM, Sui P, Wienhold MD, Sun X. Pulmonary neuroendocrine cells function as airway sensors to control lung immune response. Science 2016;351(6274):707–710.
- Cutz E, Pan J, Yeger H, Domnik NJ, Fisher JT. Recent advances and contraversies on the role of pulmonary neuroepithelial bodies as airway sensors. Semin. Cell Dev. Biol. 2013;24(1):40–50.
- Song H, Yao E, Lin C, Gacayan R, Chen M-H, Chuang P-T. Functional characterization of pulmonary neuroendocrine cells in lung development, injury, and tumorigenesis. Proceedings of the National Academy of Sciences 2012;109(43):17531–17536.
- Stahlman MT, Kasselberg AG, Orth DN, Gray ME. Ontogeny of neuroendocrine cells in human fetal lung. II. An immunohistochemical study. Lab. Invest. 1985;52(1):52–60.
- Kuo CS, Krasnow MA. Formation of a Neurosensory Organ by Epithelial Cell Slithering. Cell 2015;163(2):394–405.
- Borges M, Linnoila RI, van de Velde HJ, Chen H, Nelkin BD, Mabry M, Baylin SB, Ball DW. An achaete-scute homologue essential for neuroendocrine differentiation in the lung. Nature 1997;386(6627):852–855.
- Ito T, Udaka N, Yazawa T, Okudela K, Hayashi H, Sudo T, Guillemot F, Kageyama R, Kitamura H. Basic helix-loop-helix transcription factors regulate the neuroendocrine differentiation of fetal mouse pulmonary epithelium. Development 2000;127(18):3913–3921.
- Sunday ME. Pulmonary Neuroendocrine Cells and Lung Development. Endocr. Pathol. 1996;7(3):173–201.
- Noguchi M, Sumiyama K, Morimoto M. Directed Migration of Pulmonary Neuroendocrine Cells toward Airway Branches Organizes the Stereotypic Location of Neuroepithelial Bodies. CellReports 2015;13(12):2679–2686.
- Domyan ET, Branchfield K, Gibson DA, Naiche LA, Lewandoski M, Tessier-Lavigne M, Ma L, Sun X. Roundabout receptors are critical for foregut separation from the body wall. Dev. Cell 2013;24(1):52–63.
- Jia S, Wildner H, Birchmeier C. Insm1 controls the differentiation of pulmonary neuroendocrine cells by repressing Hes1. Dev. Biol. 2015;408(1):90–98.
- Stupnikov MR, Yang Y, Mori M, Lu J, Cardoso WV. Jagged and Delta-like ligands control distinct events during airway progenitor cell differentiation. Elife 2019;8. doi:10.7554/eLife.50487.
- Morimoto M, Nishinakamura R, Saga Y, Kopan R. Different assemblies of Notch receptors coordinate the distribution of the major bronchial Clara, ciliated and neuroendocrine cells. Development 2012;139(23):4365–4373.
- Hor P, Punj V, Calvert BA, Castaldi A, Miller AJ, Carraro G, Stripp BR, Brody SL, Spence JR, Ichida JK, Ryan Firth AL, Borok Z. Efficient Generation and Transcriptomic Profiling of Human iPSC-Derived Pulmonary Neuroendocrine Cells. iScience 2020;23(5):101083.
- Chen HJ, Poran A, Unni AM, Huang SX, Elemento O, Snoeck H-W, Varmus H. Generation of pulmonary neuro-endocrine cells and tumors resembling small cell lung cancers from human embryonic stem cells. Cold Spring Harbor Laboratory 2018:261461.
- Neptune ER, Podowski M, Calvi C, Cho J-H, Garcia JGN, Tuder R, Linnoila RI, Tsai M-J, Dietz HC. Targeted disruption of NeuroD, a proneural basic helix-loop-helix factor, impairs distal lung formation and neuroendocrine morphology in the neonatal lung. J. Biol. Chem. 2008;283(30):21160–21169.
- Reynolds SD, Giangreco A, Power JH, Stripp BR. Neuroepithelial bodies of pulmonary airways serve as a reservoir of progenitor cells capable of epithelial regeneration. Am. J. Pathol. 2000;156(1):269–278.
- Ouadah Y, Rojas ER, Riordan DP, Capostagno S, Kuo CS, Krasnow MA. Rare Pulmonary Neuroendocrine Cells Are Stem Cells Regulated by Rb, p53, and Notch. Cell 2019;179(2):403–416.e23.
- Yao E, Lin C, Wu Q, Zhang K, Song H, Chuang P-T. Notch Signaling Controls Transdifferentiation of Pulmonary Neuroendocrine Cells in Response to Lung Injury. Stem Cells 2018;36(3):377–391.
- Van Winkle LS, Gunderson AD, Shimizu JA, Baker GL, Brown CD. Gender differences in naphthalene metabolism and naphthalene-induced acute lung injury. Am. J. Physiol. Lung Cell. Mol. Physiol. 2002;282(5):L1122–34.
- Reynolds SD, Hong KU, Giangreco A, Mango GW, Guron C, Morimoto Y, Stripp BR. Conditional clara cell ablation reveals a self-renewing progenitor function of pulmonary neuroendocrine cells. American Journal of Physiology - Lung Cellular and Molecular Physiology 2000;278(6):L1256–63.
- Aguayo SM, Kane MA, King TE Jr, Schwarz MI, Grauer L, Miller YE. Increased levels of bombesin-like peptides in the lower respiratory tract of asymptomatic cigarette smokers. J. Clin. Invest. 1989;84(4):1105–1113.
- Wang G, Wang R, Ferris B, Salit J, Strulovici-Barel Y, Hackett NR, Crystal RG. Smoking-mediated up-regulation of GAD67 expression in the human airway epithelium. Respir. Res. 2010;11:150.
- Joad JP, Ji C, Kott KS, Bric JM, Pinkerton KE. In utero and postnatal effects of sidestream cigarette smoke exposure on lung function, hyperresponsiveness, and neuroendocrine cells in rats. Toxicol. Appl. Pharmacol. 1995;132(1):63–71.
- Fu XW, Spindel ER. Recruitment of GABA(A) receptors in chemoreceptor pulmonary neuroepithelial bodies by prenatal nicotine exposure in monkey lung. Adv. Exp. Med. Biol. 2009;648:439–445.
- Shivaraju M, Chitta UK, Grange RMH, Jain IH, Capen D, Liao L, Xu J, Ichinose F, Zapol WM, Mootha VK, Rajagopal J. Airway stem cells sense hypoxia and differentiate into protective solitary neuroendocrine cells. Science 2021;371(6524):52–57.
- Pan J, Yeger H, Ratcliffe P, Bishop T, Cutz E. Hyperplasia of pulmonary neuroepithelial bodies (NEB) in lungs of prolyl hydroxylase -1(PHD-1) deficient mice. Adv. Exp. Med. Biol. 2012;758:149–155.
- Ito T, Udaka N, Okudela K, Yazawa T, Kitamura H. Mechanisms of neuroendocrine differentiation in pulmonary neuroendocrine cells and small cell carcinoma. Endocr. Pathol. 2003;14(2):133–139.
- Lauweryns JM, Cokelaere M. Intrapulmonary neuro-epithelial bodies: hypoxia-sensitive neuro(chemo-)receptors. Experientia 1973;29(11):1384–1386.
- Fu XW, Nurse CA, Wong V, Cutz E. Hypoxia-induced secretion of serotonin from intact pulmonary neuroepithelial bodies in neonatal rabbit. J. Physiol. 2002;539(Pt 2):503–510.
- Livermore S, Zhou Y, Pan J, Yeger H, Nurse CA, Cutz E. Pulmonary neuroepithelial bodies are polymodal airway sensors: Evidence for CO 2/H +sensing. American Journal of Physiology - Lung Cellular and Molecular Physiology 2015;308(8):L807–L815.
- MacLean MR, Clayton RA, Templeton AG, Morecroft I. Evidence for 5-HT1-like receptor-mediated vasoconstriction in human pulmonary artery. Br. J. Pharmacol. 1996;119(2):277–282.
- Springall DR, Collina G, Barer G, Suggett AJ, Bee D, Polak JM. Increased intracellular levels of calcitonin gene-related peptide-like immunoreactivity in pulmonary endocrine cells of hypoxic rats. J. Pathol. 1988;155(3):259–267.
- Kawanami Y, Morimoto Y, Kim H, Nakamura T, Machida K, Kido T, Asonuma E, Yatera K, Yoshii C, Kido M. Calcitonin gene-related peptide stimulates proliferation of alveolar epithelial cells. Respir. Res. 2009;10:8.
- Sunday ME. Oxygen, gastrin-releasing Peptide, and pediatric lung disease: life in the balance. Front Pediatr 2014;2:72.
- Subramaniam M, Bausch C, Twomey A, Andreeva S, Yoder BA, Chang L, Crapo JD, Pierce RA, Cuttitta F, Sunday ME. Bombesin-like peptides modulate alveolarization and angiogenesis in bronchopulmonary dysplasia. Am. J. Respir. Crit. Care Med. 2007;176(9):902–912.
- Shirey KA, Sunday ME, Lai W, Patel MC, Blanco JCG, Cuttitta F, Vogel SN. Novel role of gastrin releasing peptide-mediated signaling in the host response to influenza infection. Mucosal Immunol. 2019;12(1):223–231.
- Tighe RM, Heck K, Soderblom E, Zhou S, Birukova A, Young K, Rouse D, Vidas J, Komforti MK, Toomey CB, Cuttitta F, Sunday ME. Immediate Release of Gastrin-Releasing Peptide Mediates Delayed Radiation-Induced Pulmonary Fibrosis. Am. J. Pathol. 2019;189(5):1029–1040.
- Kardon G, Ackerman KG, McCulley DJ, Shen Y, Wynn J, Shang L, Bogenschutz E, Sun X, Chung WK. Congenital diaphragmatic hernias: from genes to mechanisms to therapies. Dis. Model. Mech. 2017;10(8):955–970.
- Bousbaa H, Poron F, Fleury-Feith J. Changes in chromogranin A-immunoreactive guinea-pig pulmonary neuroendocrine cells after sensitization and challenge with ovalbumin. Cell Tissue Res. 1994;275(1):195–199.
- Sui P, Wiesner DL, Xu J, Zhang Y, Lee J, Van Dyken S, Lashua A, Yu C, Klein BS, Locksley RM, Deutsch G, Sun X. Pulmonary neuroendocrine cells amplify allergic asthma responses. Science 2018;360(6393). Available at: http://eutils.ncbi.nlm.nih.gov/entrez/eutils/elink.fcgi?dbfrom=pubmed&id=29599193&retmode=ref&cmd=prlinks.
- Nevel RJ, Garnett ET, Schaudies DA, Young LR. Growth trajectories and oxygen use in neuroendocrine cell hyperplasia of infancy. Pediatr. Pulmonol. 2018;53(5):656–663.
- Young LR, Brody AS, Inge TH, Acton JD, Bokulic RE, Langston C, Deutsch GH. Neuroendocrine cell distribution and frequency distinguish neuroendocrine cell hyperplasia of infancy from other pulmonary disorders. Chest 2011;139(5):1060–1071.
- Nevel RJ, Garnett ET, Worrell JA, Morton RL, Nogee LM, Blackwell TS, Young LR. Persistent Lung Disease in Adults with NKX2.1 Mutation and Familial Neuroendocrine Cell Hyperplasia of Infancy. Ann. Am. Thorac. Soc. 2016;13(8):1299–1304.
- Deterding RR, Pye C, Fan LL, Langston C. Persistent tachypnea of infancy is associated with neuroendocrine cell hyperplasia. Pediatr. Pulmonol. 2005;40(2):157–165.
- Popler J, Gower WA, Mogayzel PJ Jr, Nogee LM, Langston C, Wilson AC, Hay TC, Deterding RR. Familial neuroendocrine cell hyperplasia of infancy. Pediatr. Pulmonol. 2010;45(8):749–755.
- Young LR, Deutsch GH, Bokulic RE, Brody AS, Nogee LM. A mutation in TTF1/NKX2.1 is associated with familial neuroendocrine cell hyperplasia of infancy. Chest 2013;144(4):1199–1206.
- Davies SJ, Gosney JR, Hansell DM, Wells AU, du Bois RM, Burke MM, Sheppard MN, Nicholson AG. Diffuse idiopathic pulmonary neuroendocrine cell hyperplasia: an under-recognised spectrum of disease. Thorax 2007;62(3):248–252.
- Nassar AA, Jaroszewski DE, Helmers RA, Colby TV, Patel BM, Mookadam F. Diffuse idiopathic pulmonary neuroendocrine cell hyperplasia: a systematic overview. Am. J. Respir. Crit. Care Med. 2011;184(1):8–16.
- Rossi G, Cavazza A, Spagnolo P, Sverzellati N, Longo L, Jukna A, Montanari G, Carbonelli C, Vincenzi G, Bogina G, Franco R, Tiseo M, Cottin V, Colby TV. Diffuse idiopathic pulmonary neuroendocrine cell hyperplasia syndrome. Eur. Respir. J. 2016;47(6):1829–1841.
- Warth A, Herpel E, Schmähl A, Storz K, Schnabel PA. Diffuse idiopathic pulmonary neuroendocrine cell hyperplasia (DIPNECH) in association with an adenocarcinoma: a case report. J. Med. Case Rep. 2008;2:21.
- Mireskandari M, Abdirad A, Zhang Q, Dietel M, Petersen I. Association of small foci of diffuse idiopathic pulmonary neuroendocrine cell hyperplasia (DIPNECH) with adenocarcinoma of the lung. Pathol. Res. Pract. 2013;209(9):578–584.
- Escudero AG, Zarco ER, Arjona JCG, Moreno MJR, Rodríguez KG, Benítez AV, Cámpora RG. Expression of developing neural transcription factors in diffuse idiopathic pulmonary neuroendocrine cell hyperplasia (DIPNECH). Virchows Arch. 2016;469(3):357–363.
- Gosney JR, Williams IJ, Dodson AR, Foster CS. Morphology and antigen expression profile of pulmonary neuroendocrine cells in reactive proliferations and diffuse idiopathic pulmonary neuroendocrine cell hyperplasia (DIPNECH). Histopathology 2011;59(4):751–762.
- Flint K, Ye C, Henry TL. Diffuse Idiopathic Pulmonary Neuroendocrine Cell Hyperplasia (DIPNECH) with liver metastases. BMJ Case Rep. 2019;12(6). doi:10.1136/bcr-2018-228536.
- Carr LL, Chung JH, Duarte Achcar R, Lesic Z, Rho JY, Yagihashi K, Tate RM, Swigris JJ, Kern JA. The clinical course of diffuse idiopathic pulmonary neuroendocrine cell hyperplasia. Chest 2015;147(2):415–422.
- Chauhan A, Ramirez RA. Diffuse Idiopathic Pulmonary Neuroendocrine Cell Hyperplasia (DIPNECH) and the Role of Somatostatin analogs: A Case Series. Lung 2015;193(5):653–657.
- Al-Toubah T, Strosberg J, Halfdanarson TR, Oleinikov K, Gross DJ, Haider M, Sonbol MB, Almquist D, Grozinsky-Glasberg S. Somatostatin Analogs Improve Respiratory Symptoms in Patients With Diffuse Idiopathic Neuroendocrine Cell Hyperplasia. Chest 2020;158(1):401–405.
- Rindi G, Wiedenmann B. Neuroendocrine neoplasms of the gut and pancreas: New insights. Nature Reviews Endocrinology 2012;8(1):54–64.
- Pusceddu S, Vitali M, Haspinger E, Tavecchio L, Giovannetti R, Bille A, Concas L, Garassino M, Milione M, Braud F de, Buzzoni R. Update on therapeutic strategy in lung carcinoids. J. Cancer Ther. 2013;04(10):1466–1471.
- Travis WD. Advances in neuroendocrine lung tumors. Ann. Oncol. 2010;21(Supplement 7):vii65–vii71.
- Rindi G, Klimstra DS, Abedi-Ardekani B, Asa SL, Bosman FT, Brambilla E, Busam KJ, Krijger RR, Dietel M, El-Naggar AK, Fernandez-Cuesta L, Klöppel G, McCluggage WG, Moch H, Ohgaki H, Rakha EA, Reed NS, Rous BA, Sasano H, Scarpa A, Scoazec J-Y, Travis WD, Tallini G, Trouillas J, Krieken JH, Cree IA. A common classification framework for neuroendocrine neoplasms: an International Agency for Research on Cancer (IARC) and World Health Organization (WHO) expert consensus proposal. Mod. Pathol. 2018:1–17.
- Rossi G, Bertero L, Marchiò C, Papotti M. Molecular alterations of neuroendocrine tumours of the lung. Histopathology 2018;72(1):142–152.
- Soomro Z, Youssef M, Yust-Katz S, Jalali A, Patel AJ, Mandel J. Paraneoplastic syndromes in small cell lung cancer. J. Thorac. Dis. 2020;12(10):6253–6263.
- Gazdar AF, Girard L, Lockwood WW, Lam WL, Minna JD. Lung cancer cell lines as tools for biomedical discovery and research. J. Natl. Cancer Inst. 2010;102(17):1310–1321.
- Drapkin BJ, George J, Christensen CL, Mino-Kenudson M, Dries R, Sundaresan T, Phat S, Myers DT, Zhong J, Igo P, Hazar-Rethinam MH, Licausi JA, Gomez-Caraballo M, Kem M, Jani KN, Azimi R, Abedpour N, Menon R, Lakis S, Heist RS, Büttner R, Haas S, Sequist LV, Shaw AT, Wong K-K, Hata AN, Toner M, Maheswaran S, Haber DA, Peifer M, Dyson N, Thomas RK, Farago AF. Genomic and Functional Fidelity of Small Cell Lung Cancer Patient-Derived Xenografts. Cancer Discov. 2018;8(5):600–615.
- Gazdar AF, Savage TK, Johnson JE, Berns A, Sage J, Linnoila RI, MacPherson D, McFadden DG, Farago A, Jacks T, Travis WD, Brambilla E. The comparative pathology of genetically engineered mouse models for neuroendocrine carcinomas of the lung. J. Thorac. Oncol. 2015;10(4):553–564.
- George J, Lim JS, Jang SJ, Cun Y, Ozretić L, Kong G, Leenders F, Lu X, Fernández-Cuesta L, Bosco G, Müller C, Dahmen I, Jahchan NS, Park K-S, Yang D, Karnezis AN, Vaka D, Torres A, Wang MS, Korbel JO, Menon R, Chun S-M, Kim D, Wilkerson M, Hayes N, Engelmann D, Pützer B, Bos M, Michels S, Vlasic I, Seidel D, Pinther B, Schaub P, Becker C, Altmüller J, Yokota J, Kohno T, Iwakawa R, Tsuta K, Noguchi M, Muley T, Hoffmann H, Schnabel PA, Petersen I, Chen Y, Soltermann A, Tischler V, Choi C-M, Kim Y-H, Massion PP, Zou Y, Jovanovic D, Kontic M, Wright GM, Russell PA, Solomon B, Koch I, Lindner M, Muscarella LA, la Torre A, Field JK, Jakopovic M, Knezevic J, Castaños-Vélez E, Roz L, Pastorino U, Brustugun O-T, Lund-Iversen M, Thunnissen E, Köhler J, Schuler M, Botling J, Sandelin M, Sanchez-Cespedes M, Salvesen HB, Achter V, Lang U, Bogus M, Schneider PM, Zander T, Ansén S, Hallek M, Wolf J, Vingron M, Yatabe Y, Travis WD, Nürnberg P, Reinhardt C, Perner S, Heukamp L, Büttner R, Haas SA, Brambilla E, Peifer M, Sage J, Thomas RK. Comprehensive genomic profiles of small cell lung cancer. Nature 2015;524(7563):47–53.
- Meuwissen R, Linn SC, Linnoila RI, Zevenhoven J, Mooi WJ, Berns A. Induction of small cell lung cancer by somatic inactivation of both Trp53 and Rb1 in a conditional mouse model. Cancer Cell 2003;4(3):181–189.
- Peifer M, Fernandez-Cuesta L, Sos ML, George J, Seidel D, Kasper LH, Plenker D, Leenders F, Sun R, Zander T, Menon R, Koker M, Dahmen I, Müller C, Di Cerbo V, Schildhaus H-U, Altmüller J, Baessmann I, Becker C, de Wilde B, Vandesompele J, Böhm D, Ansén S, Gabler F, Wilkening I, Heynck S, Heuckmann JM, Lu X, Carter SL, Cibulskis K, Banerji S, Getz G, Park K-S, Rauh D, Grütter C, Fischer M, Pasqualucci L, Wright G, Wainer Z, Russell P, Petersen I, Chen Y, Stoelben E, Ludwig C, Schnabel P, Hoffmann H, Muley T, Brockmann M, Engel-Riedel W, Muscarella LA, Fazio VM, Groen H, Timens W, Sietsma H, Thunnissen E, Smit E, Heideman DAM, Snijders PJF, Cappuzzo F, Ligorio C, Damiani S, Field J, Solberg S, Brustugun OT, Lund-Iversen M, Sänger J, Clement JH, Soltermann A, Moch H, Weder W, Solomon B, Soria J-C, Validire P, Besse B, Brambilla E, Brambilla C, Lantuejoul S, Lorimier P, Schneider PM, Hallek M, Pao W, Meyerson M, Sage J, Shendure J, Schneider R, Büttner R, Wolf J, Nürnberg P, Perner S, Heukamp LC, Brindle PK, Haas S, Thomas RK. Integrative genome analyses identify key somatic driver mutations of small-cell lung cancer. Nat. Genet. 2012;44(10):1104–1110.
- McFadden DG, Papagiannakopoulos T, Taylor-Weiner A, Stewart C, Carter SL, Cibulskis K, Bhutkar A, McKenna A, Dooley A, Vernon A, Sougnez C, Malstrom S, Heimann M, Park J, Chen F, Farago AF, Dayton T, Shefler E, Gabriel S, Getz G, Jacks T. Genetic and clonal dissection of murine small cell lung carcinoma progression by genome sequencing. Cell 2014;156(6):1298–1311.
- Sutherland KD, Proost N, Brouns I, Adriaensen D, Song J-Y, Berns A. Cell of Origin of Small Cell Lung Cancer: Inactivation of Trp53 and Rb1 in Distinct Cell Types of Adult Mouse Lung. Cancer Cell 2011;19(6):754–764.
- Yang D, Denny SK, Greenside PG, Chaikovsky AC, Brady JJ, Ouadah Y, Granja JM, Jahchan NS, Lim JS, Kwok S, Kong CS, Berghoff AS, Schmitt A, Reinhardt HC, Park K-S, Preusser M, Kundaje A, Greenleaf WJ, Sage J, Winslow MM. Intertumoral Heterogeneity in SCLC Is Influenced by the Cell Type of Origin. Cancer Discov. 2018;8(10):1316–1331.
- Dooley AL, Winslow MM, Chiang DY, Banerji S, Stransky N, Dayton TL, Snyder EL, Senna S, Whittaker CA, Bronson RT, Crowley D, Barretina J, Garraway L, Meyerson M, Jacks T. Nuclear factor I/B is an oncogene in small cell lung cancer. Genes Dev. 2011;25(14):1470–1475.
- Denny SK, Yang D, Chuang C-H, Brady JJ, Lim JS, Grüner BM, Chiou S-H, Schep AN, Baral J, Hamard C, Antoine M, Wislez M, Kong CS, Connolly AJ, Park K-S, Sage J, Greenleaf WJ, Winslow MM. Nfib Promotes Metastasis through a Widespread Increase in Chromatin Accessibility. Cell 2016;166(2):328–342.
- Semenova EA, Kwon M-C, Monkhorst K, Song J-Y, Bhaskaran R, Krijgsman O, Kuilman T, Peters D, Buikhuisen WA, Smit EF, Pritchard C, Cozijnsen M, van der Vliet J, Zevenhoven J, Lambooij J-P, Proost N, van Montfort E, Velds A, Huijbers IJ, Berns A. Transcription Factor NFIB Is a Driver of Small Cell Lung Cancer Progression in Mice and Marks Metastatic Disease in Patients. CellReports 2016;16(3):631–643.
- Mollaoglu G, Guthrie MR, Böhm S, Brägelmann J, Can I, Ballieu PM, Marx A, George J, Heinen C, Chalishazar MD, Cheng H, Ireland AS, Denning KE, Mukhopadhyay A, Vahrenkamp JM, Berrett KC, Mosbruger TL, Wang J, Kohan JL, Salama ME, Witt BL, Peifer M, Thomas RK, Gertz J, Johnson JE, Gazdar AF, Wechsler-Reya RJ, Sos ML, Oliver TG. MYC Drives Progression of Small Cell Lung Cancer to a Variant Neuroendocrine Subtype with Vulnerability to Aurora Kinase Inhibition. Cancer Cell 2016:1–17.
- Rudin CM, Poirier JT, Byers LA, Dive C, Dowlati A, George J, Heymach JV, Johnson JE, Lehman JM, MacPherson D, Massion PP, Minna JD, Oliver TG, Quaranta V, Sage J, Thomas RK, Vakoc CR, Gazdar AF. Molecular subtypes of small cell lung cancer: a synthesis of human and mouse model data. Nat. Rev. Cancer 2019;19(5):289–297.
- Gay CM, Stewart CA, Park EM, Diao L, Groves SM, Heeke S, Nabet BY, Fujimoto J, Solis LM, Lu W, Xi Y, Cardnell RJ, Wang Q, Fabbri G, Cargill KR, Vokes NI, Ramkumar K, Zhang B, Della Corte CM, Robson P, Swisher SG, Roth JA, Glisson BS, Shames DS, Wistuba II, Wang J, Quaranta V, Minna J, Heymach JV, Byers LA. Patterns of transcription factor programs and immune pathway activation define four major subtypes of SCLC with distinct therapeutic vulnerabilities. Cancer Cell 2021. doi:10.1016/j.ccell.2020.12.014.
- Huang Y-H, Klingbeil O, He X-Y, Wu XS, Arun G, Lu B, Somerville TDD, Milazzo JP, Wilkinson JE, Demerdash OE, Spector DL, Egeblad M, Shi J, Vakoc CR. POU2F3 is a master regulator of a tuft cell-like variant of small cell lung cancer. Genes Dev. 2018;32(13-14):915–928.
- Ireland AS, Micinski AM, Kastner DW, Guo B, Wait SJ, Spainhower KB, Conley CC, Chen OS, Guthrie MR, Soltero D, Qiao Y, Huang X, Tarapcsák S, Devarakonda S, Chalishazar MD, Gertz J, Moser JC, Marth G, Puri S, Witt BL, Spike BT, Oliver TG. MYC Drives Temporal Evolution of Small Cell Lung Cancer Subtypes by Reprogramming Neuroendocrine Fate. Cancer Cell 2020;38(1):60–78.e12.
- Poirier JT, George J, Owonikoko TK, Berns A, Brambilla E, Byers LA, Carbone D, Chen HJ, Christensen CL, Dive C, Farago AF, Govindan R, Hann C, Hellmann MD, Horn L, Johnson JE, Ju YS, Kang S, Krasnow M, Lee J, Lee S-H, Lehman J, Lok B, Lovly C, MacPherson D, McFadden D, Minna J, Oser M, Park K, Park K-S, Pommier Y, Quaranta V, Ready N, Sage J, Scagliotti G, Sos ML, Sutherland KD, Travis WD, Vakoc CR, Wait SJ, Wistuba I, Wong KK, Zhang H, Daigneault J, Wiens J, Rudin CM, Oliver TG. New Approaches to SCLC Therapy: From the Laboratory to the Clinic. J. Thorac. Oncol. 2020;15(4):520–540.
- Stewart CA, Gay CM, Xi Y, Sivajothi S, Sivakamasundari V, Fujimoto J, Bolisetty M, Hartsfield PM, Balasubramaniyan V, Chalishazar MD, Moran C, Kalhor N, Stewart J, Tran H, Swisher SG, Roth JA, Zhang J, de Groot J, Glisson B, Oliver TG, Heymach JV, Wistuba I, Robson P, Wang J, Byers LA. Single-cell analyses reveal increased intratumoral heterogeneity after the onset of therapy resistance in small-cell lung cancer. Nat Cancer 2020;1:423–436.
- Calbo J, van Montfort E, Proost N, van Drunen E, Beverloo HB, Meuwissen R, Berns A. A functional role for tumor cell heterogeneity in a mouse model of small cell lung cancer. Cancer Cell 2011;19(2):244–256.
- Böttger F, Semenova EA, Song J-Y, Ferone G, van der Vliet J, Cozijnsen M, Bhaskaran R, Bombardelli L, Piersma SR, Pham TV, Jimenez CR, Berns A. Tumor Heterogeneity Underlies Differential Cisplatin Sensitivity in Mouse Models of Small-Cell Lung Cancer. Cell Rep. 2019;27(11):3345–3358.e4.
- Lim JS, Ibaseta A, Fischer MM, Cancilla B, O’Young G, Cristea S, Luca VC, Yang D, Jahchan NS, Hamard C, Antoine M, Wislez M, Kong C, Cain J, Liu Y-W, Kapoun AM, Garcia KC, Hoey T, Murriel CL, Sage J. Intratumoural heterogeneity generated by Notch signalling promotes small-cell lung cancer. Nature 2017:1–22.
- Jahchan NS, Dudley JT, Mazur PK, Flores N, Yang D, Palmerton A, Zmoos A-F, Vaka D, Tran KQT, Zhou M, Krasinska K, Riess JW, Neal JW, Khatri P, Park KS, Butte AJ, Sage J. A drug repositioning approach identifies tricyclic antidepressants as inhibitors of small cell lung cancer and other neuroendocrine tumors. Cancer Discov. 2013;3(12):1364–1377.
- Li L, Ng SR, Colón CI, Drapkin BJ, Hsu PP, Li Z, Nabel CS, Lewis CA, Romero R, Mercer KL, Bhutkar A, Phat S, Myers DT, Muzumdar MD, Westcott PMK, Beytagh MC, Farago AF, Vander Heiden MG, Dyson NJ, Jacks T. Identification of DHODH as a therapeutic target in small cell lung cancer. Sci. Transl. Med. 2019;11(517):eaaw7852.
- Coles GL, Cristea S, Webber JT, Levin RS, Moss SM, He A, Sangodkar J, Hwang YC, Arand J, Drainas AP, Mooney NA, Demeter J, Spradlin JN, Mauch B, Le V, Shue YT, Ko JH, Lee MC, Kong C, Nomura DK, Ohlmeyer M, Swaney DL, Krogan NJ, Jackson PK, Narla G, Gordan JD, Shokat KM, Sage J. Unbiased Proteomic Profiling Uncovers a Targetable GNAS/PKA/PP2A Axis in Small Cell Lung Cancer Stem Cells. Cancer Cell 2020;38(1):129–143.e7.
- Lantuejoul S, Fernandez-Cuesta L, Damiola F, Girard N, McLeer A. New molecular classification of large cell neuroendocrine carcinoma and small cell lung carcinoma with potential therapeutic impacts. Transl Lung Cancer Res 2020;9(5):2233–2244.
- Derks JL, van Suylen RJ, Thunnissen E, den Bakker MA, Groen HJ, Smit EF, Damhuis RA, van den Broek EC, Speel E-JM, Dingemans A-MC, PALGA group. Chemotherapy for pulmonary large cell neuroendocrine carcinomas: does the regimen matter? Eur. Respir. J. 2017;49(6). doi:10.1183/13993003.01838-2016.
- Akeno N, Reece AL, Callahan M, Miller AL, Kim RG, He D, Lane A, Moulton JS, Wikenheiser-Brokamp KA. TRP53 Mutants Drive Neuroendocrine Lung Cancer Through Loss-of-Function Mechanisms with Gain-of-Function Effects on Chemotherapy Response. Mol. Cancer Ther. 2017;16(12):2913–2926.
- Lázaro S, Pérez-Crespo M, Lorz C, Bernardini A, Oteo M, Enguita AB, Romero E, Hernández P, Tomás L, Morcillo MÁ, Paramio JM, Santos M. Differential development of large-cell neuroendocrine or small-cell lung carcinoma upon inactivation of 4 tumor suppressor genes. Proc. Natl. Acad. Sci. U. S. A. 2019;116(44):22300–22306.
- George J, Walter V, Peifer M, Alexandrov LB, Seidel D, Leenders F, Maas L, Müller C, Dahmen I, Delhomme TM, Ardin M, Leblay N, Byrnes G, Sun R, Reynies A, McLeer-Florin A, Bosco G, Malchers F, Menon R, Altmüller J, Becker C, Nürnberg P, Achter V, Lang U, Schneider PM, Bogus M, Soloway MG, Wilkerson MD, Cun Y, McKay JD, Moro-Sibilot D, Brambilla CG, Lantuejoul S, Lemaitre N, Soltermann A, Weder W, Tischler V, Brustugun OT, Lund-Iversen M, Helland Å, Solberg S, Ansén S, Wright G, Solomon B, Roz L, Pastorino U, Petersen I, Clement JH, Sänger J, Wolf J, Vingron M, Zander T, Perner S, Travis WD, Haas SA, Olivier M, Foll M, Büttner R, Hayes DN, Brambilla E, Fernandez-Cuesta L, Thomas RK. Integrative genomic profiling of large-cell neuroendocrine carcinomas reveals distinct subtypes of high-grade neuroendocrine lung tumors. Nat. Commun. 2018:1–13.
- Derks JL, Leblay N, Thunnissen E, van Suylen RJ, den Bakker M, Groen HJM, Smit EF, Damhuis R, van den Broek EC, Charbrier A, Foll M, McKay JD, Fernandez-Cuesta L, Speel E-JM, Dingemans A-MC, PALGA-Group. Molecular Subtypes of Pulmonary Large-cell Neuroendocrine Carcinoma Predict Chemotherapy Treatment Outcome. Clin. Cancer Res. 2018;24(1):33–42.
- Hermans BCM, Derks JL, Thunnissen E, van Suylen RJ, den Bakker MA, Groen HJM, Smit EF, Damhuis RA, van den Broek EC, PALGA-group, Ruland A, Speel EJM, Dingemans AMC. DLL3 expression in large cell neuroendocrine carcinoma (LCNEC) and association with molecular subtypes and neuroendocrine profile. Lung Cancer 2019;138:102–108.
- Miyoshi T, Umemura S, Matsumura Y, Mimaki S, Tada S, Makinoshima H, Ishii G, Udagawa H, Matsumoto S, Yoh K, Niho S, Ohmatsu H, Aokage K, Hishida T, Yoshida J, Nagai K, Goto K, Tsuboi M, Tsuchihara K. Genomic Profiling of Large-Cell Neuroendocrine Carcinoma of the Lung. Clin. Cancer Res. 2017;23(3):757–765.
- Rekhtman N, Pietanza MC, Hellmann MD, Naidoo J, Arora A, Won H, Halpenny DF, Wang H, Tian SK, Litvak AM, Paik PK, Drilon AE, Socci N, Poirier JT, Shen R, Berger MF, Moreira AL, Travis WD, Rudin CM, Ladanyi M. Next-Generation Sequencing of Pulmonary Large Cell Neuroendocrine Carcinoma Reveals Small Cell Carcinoma–like and Non–Small Cell Carcinoma–like Subsets. Clin. Cancer Res. 2016;22(14):3618–3629.
- Lou G, Yu X, Song Z. Molecular Profiling and Survival of Completely Resected Primary Pulmonary Neuroendocrine Carcinoma. Clin. Lung Cancer 2017;18(3):e197–e201.
- Derks JL, Leblay N, Lantuejoul S, Dingemans A-MC, Speel E-JM, Fernandez-Cuesta L. New Insights into the Molecular Characteristics of Pulmonary Carcinoids and Large Cell Neuroendocrine Carcinomas, and the Impact on Their Clinical Management. J. Thorac. Oncol. 2018;13(6):752–766.
- Modlin IM, Kidd M, Filosso PL, Roffinella M, Lewczuk A, Cwikla J, Bodei L, Kolasinska-Cwikla A, Chung K-M, Tesselaar ME, Drozdov IA. Molecular strategies in the management of bronchopulmonary and thymic neuroendocrine neoplasms. J. Thorac. Dis. 2017;9(S15):S1458–S1473.
- Metovic J, Barella M, Bianchi F, Hofman P, Hofman V, Remmelink M, Kern I, Carvalho L, Pattini L, Sonzogni A, Veronesi G, Harari S, Forest F, Papotti M, Pelosi G. Morphologic and molecular classification of lung neuroendocrine neoplasms. Virchows Arch. 2021;478(1):5–19.
- Baudin E, Hayes AR, Scoazec J-Y, Filosso PL, Lim E, Kaltsas G, Frilling A, Chen J, Kos-Kudła B, Gorbunova V, Wiedenmann B, Nieveen van Dijkum E, Ćwikła JB, Falkerby J, Valle JW, Kulke MH, Caplin ME, ENETS 2016 Munich Advisory Board Participants, ENETS 2016 Munich Advisory Board Participants. Unmet Medical Needs in Pulmonary Neuroendocrine (Carcinoid) Neoplasms. Neuroendocrinology 2019;108(1):7–17.
- Terra Md SBSP, Xie Md PhD H, Boland Md JM, Mansfield Md AS, Molina Md PhD JR, Roden Md AC. Loss of ATRX expression predicts worse prognosis in pulmonary carcinoid tumors. Hum. Pathol. 2019;94:78–85.
- Baine MK, Rekhtman N. Multiple faces of pulmonary large cell neuroendocrine carcinoma: update with a focus on practical approach to diagnosis. Transl Lung Cancer Res 2020;9(3):860–878.
- Moonen L, Derks J, Dingemans A-M, Speel E-J. Orthopedia Homeobox (OTP) in Pulmonary Neuroendocrine Tumors: The Diagnostic Value and Possible Molecular Interactions. Cancers2019;11(10). doi:10.3390/cancers11101508.
- Lou F, Sarkaria I, Pietanza C, Travis W, Roh MS, Sica G, Healy D, Rusch V, Huang J. Recurrence of pulmonary carcinoid tumors after resection: implications for postoperative surveillance. Ann. Thorac. Surg. 2013;96(4):1156–1162.
- Cañizares MA, Matilla JM, Cueto A, Algar J, Muguruza I, Moreno-Mata N, Moreno-Balsalobre R, Guijarro R, Arrabal R, Garcia-Fontan E, Gonzalez-Piñeiro A, Garcia-Yuste M, EMETNE-SEPAR Members. Atypical carcinoid tumours of the lung: prognostic factors and patterns of recurrence. Thorax 2014;69(7):648–653.
- Gridelli C, Rossi A, Airoma G, Bianco R, Costanzo R, Daniele B, Chiara GD, Grimaldi G, Irtelli L, Maione P, Morabito A, Piantedosi FV, Riccardi F. Treatment of pulmonary neuroendocrine tumours: state of the art and future developments. Cancer Treat. Rev. 2013;39(5):466–472.
- Kneuertz PJ, Kamel MK, Stiles BM, Lee BE, Rahouma M, Harrison SW, Altorki NK, Port JL. Incidence and Prognostic Significance of Carcinoid Lymph Node Metastases. Ann. Thorac. Surg. 2018;106(4):981–988.
- Steuer CE, Behera M, Kim S, Chen Z, Saba NF, Pillai RN, Owonikoko TK, Khuri FR, Ramalingam SS. Atypical carcinoid tumor of the lung: a surveillance, epidemiology, and end results database analysis. J. Thorac. Oncol. 2015;10(3):479–485.
- Yao JC, Fazio N, Singh S, Buzzoni R, Carnaghi C, Wolin E, Tomasek J, Raderer M, Lahner H, Voi M, Pacaud LB, Rouyrre N, Sachs C, Valle JW, Fave GD, Van Cutsem E, Tesselaar M, Shimada Y, Oh D-Y, Strosberg J, Kulke MH, Pavel ME, RAD001 in Advanced Neuroendocrine Tumours, Fourth Trial (RADIANT-4) Study Group. Everolimus for the treatment of advanced, non-functional neuroendocrine tumours of the lung or gastrointestinal tract (RADIANT-4): a randomised, placebo-controlled, phase 3 study. Lancet 2016;387(10022):968–977.
- Lorz C, Oteo M, Santos M. Neuroendocrine Lung Cancer Mouse Models: An Overview. Cancers2020;13(1). doi:10.3390/cancers13010014.
- Lázaro S, Pérez-Crespo M, Enguita AB, Hernández P, Martínez-Palacio J, Oteo M, Sage J, Paramio JM, Santos M. Ablating all three retinoblastoma family members in mouse lung leads to neuroendocrine tumor formation. Oncotarget 2017;8(3):4373–4386.
- Fernandez-Cuesta L, Peifer M, Lu X, Sun R, Cacute LO, Seidel D, Zander T, Leenders F, George J, Ller CMU, Dahmen I, Pinther B, Bosco G, Konrad K, Ller JAU, Rnberg PNU, Achter V, Lang U, Schneider PM, Bogus M, Soltermann A, Brustugun OT, Helland AS, Solberg S, Lund-Iversen M, N SAE, Stoelben E, Wright GM, Russell P, Wainer Z, Solomon B, Field JK, Hyde R, Davies MPA, Heukamp LC, Petersen I, Perner S, Lovly CM, Cappuzzo F, Travis WD, Wolf JUR, Vingron M, Brambilla E, Haas SA, Buettner R, Thomas RK. Frequent mutations in chromatin-remodelling genes in pulmonary carcinoids. Nat. Commun. 2014;5:1–7.
- Simbolo M, Mafficini A, Sikora KO, Fassan M, Barbi S, Corbo V, Mastracci L, Rusev B, Grillo F, Vicentini C, Ferrara R, Pilotto S, Davini F, Pelosi G, Lawlor RT, Chilosi M, Tortora G, Bria E, Fontanini G, Volante M, Scarpa A. Lung neuroendocrine tumours: deep sequencing of the four World Health Organization histotypes reveals chromatin-remodelling genes as major players and a prognostic role for TERT, RB1, MEN1 and KMT2D. J. Pathol. 2017;241(4):488–500.
- de Laat JM, Pieterman CR, van den Broek MF, Twisk JW, Hermus AR, Dekkers OM, de Herder WW, van der Horst-Schrivers AN, Drent ML, Bisschop PH, Havekes B, Vriens MR, Valk GD. Natural course and survival of neuroendocrine tumors of thymus and lung in MEN1 patients. J. Clin. Endocrinol. Metab. 2014;99(9):3325–3333.
- Voortman J, Lee J-H, Killian JK, Suuriniemi M, Wang Y, Lucchi M, Smith WI Jr, Meltzer P, Wang Y, Giaccone G. Array comparative genomic hybridization-based characterization of genetic alterations in pulmonary neuroendocrine tumors. Proc. Natl. Acad. Sci. U. S. A. 2010;107(29):13040–13045.
- Alcala N, Leblay N, Gabriel AAG, Mangiante L, Hervas D, Giffon T, Sertier AS, Ferrari A, Derks J, Ghantous A, Delhomme TM, Chabrier A, Cuenin C, Abedi-Ardekani B, Boland A, Olaso R, Meyer V, Altmuller J, Calvez-Kelm F, Durand G, Voegele C, Boyault S, Moonen L, Lemaitre N, Lorimier P, Toffart AC, Soltermann A, Clement JH, Saenger J, Field JK, Brevet M, Blanc-Fournier C, Galateau-Salle F, Le Stang N, Russell PA, Wright G, Sozzi G, Pastorino U, Lacomme S, Vignaud JM, Hofman V, Hofman P, Brustugun OT, Lund-Iversen M, de Montpreville VT, Muscarella LA, Graziano P, Popper H, Stojsic J, Deleuze JF, Herceg Z, Viari A, Nuernberg P, Pelosi G, Dingemans AMC, Milione M, Roz L, Brcic L, Volante M, Papotti MG, Caux C, Sandoval J, Hernandez-Vargas H, Brambilla E, Speel EJM, Girard N, Lantuejoul S, McKay JD, Foll M, Fernandez-Cuesta L. Integrative and comparative genomic analyses identify clinically relevant pulmonary carcinoid groups and unveil the supra-carcinoids. Nat. Commun. 2019:1–21.
- Laddha SV, da Silva EM, Robzyk K, Untch BR, Ke H, Rekhtman N, Poirier JT, Travis WD, Tang LH, Chan CS. Integrative Genomic Characterization Identifies Molecular Subtypes of Lung Carcinoids. Cancer Res. 2019;79(17):4339–4347.
- Gabriel AAG, Mathian E, Mangiante L, Voegele C, Cahais V, Ghantous A, McKay JD, Alcala N, Fernandez-Cuesta L, Foll M. A molecular map of lung neuroendocrine neoplasms. Gigascience 2020;9(11). doi:10.1093/gigascience/giaa112.
- Fernandez-Cuesta L, Foll M. Molecular studies of lung neuroendocrine neoplasms uncover new concepts and entities. Transl Lung Cancer Res 2019;8(Suppl 4):S430–S434.
- Simbolo M, Barbi S, Fassan M, Mafficini A, Ali G, Vicentini C, Sperandio N, Corbo V, Rusev B, Mastracci L, Grillo F, Pilotto S, Pelosi G, Pelliccioni S, Lawlor RT, Tortora G, Fontanini G, Volante M, Scarpa A, Bria E. Gene Expression Profiling of Lung Atypical Carcinoids and Large Cell Neuroendocrine Carcinomas Identifies Three Transcriptomic Subtypes with Specific Genomic Alterations. J. Thorac. Oncol. 2019;14(9):1651–1661.
- Marchiò C, Gatti G, Massa F, Bertero L, Filosso P, Pelosi G, Cassoni P, Volante M, Papotti M. Distinctive pathological and clinical features of lung carcinoids with high proliferation index. Virchows Arch. 2017;471(6):713–720.
- Rindi G, Klersy C, Inzani F, Fellegara G, Ampollini L, Ardizzoni A, Campanini N, Carbognani P, De Pas TM, Galetta D, Granone PL, Righi L, Rusca M, Spaggiari L, Tiseo M, Viale G, Volante M, Papotti M, Pelosi G. Grading the neuroendocrine tumors of the lung: an evidence-based proposal. Endocr. Relat. Cancer 2014;21(1):1–16.
- Pelosi G, Papotti M, Rindi G, Scarpa A. Unraveling tumor grading and genomic landscape in lung neuroendocrine tumors. Endocr. Pathol. 2014;25(2):151–164.
- Quinn AM, Chaturvedi A, Nonaka D. High-grade Neuroendocrine Carcinoma of the Lung With Carcinoid Morphology: A Study of 12 Cases. Am. J. Surg. Pathol. 2017;41(2):263–270.
- Rekhtman N, Desmeules P, Litvak AM, Pietanza MC, Santos-Zabala ML, Ni A, Montecalvo J, Chang JC, Beras A, Preeshagul IR, Sabari JK, Rudin CM, Ladanyi M, Klimstra DS, Travis WD, Lai W-C. Stage IV lung carcinoids: spectrum and evolution of proliferation rate, focusing on variants with elevated proliferation indices. Mod. Pathol. 2019;32(8):1106–1122.
- Megyesi M, Berta M, Khoor A. Endobronchial large cell neuroendocrine carcinoma. Pathol. Oncol. Res. 2003;9(3):198–200.
- van der Flier LG, Clevers H. Stem cells, self-renewal, and differentiation in the intestinal epithelium. Annu. Rev. Physiol. 2009;71:241–260.
- Ahlman H, Nilsson. The gut as the largest endocrine organ in the body. Ann. Oncol. 2001;12 Suppl 2:S63–8.
- Baeyens L, Lemper M, Staels W, De Groef S, De Leu N, Heremans Y, German MS, Heimberg H. (re)generating human beta cells: Status, pitfalls, and perspectives. Physiol. Rev. 2018;98(3):1143–1167.
- Ionescu-Tirgoviste C, Gagniuc PA, Gubceac E, Mardare L, Popescu I, Dima S, Militaru M. A 3D map of the islet routes throughout the healthy human pancreas. Sci. Rep. 2015;5:14634.
- Schepers A, Clevers H. Wnt signaling, stem cells, and cancer of the gastrointestinal tract. Cold Spring Harb. Perspect. Biol. 2012;4(4):a007989.
- Soybel DI. Anatomy and physiology of the stomach. Surg. Clin. North Am. 2005;85(5):875–94, v.
- Kim T-H, Shivdasani RA. Stomach development, stem cells and disease. Development 2016;143(4):554–565.
- Han S, Fink J, Jörg DJ, Lee E, Yum MK, Chatzeli L, Merker SR, Josserand M, Trendafilova T, Andersson-Rolf A, Dabrowska C, Kim H, Naumann R, Lee J-H, Sasaki N, Mort RL, Basak O, Clevers H, Stange DE, Philpott A, Kim JK, Simons BD, Koo B-K. Defining the Identity and Dynamics of Adult Gastric Isthmus Stem Cells. Cell Stem Cell 2019;25(3):342–356.e7.
- Fakhry J, Stebbing MJ, Hunne B, Bayguinov Y, Ward SM, Sasse KC, Callaghan B, McQuade RM, Furness JB. Relationships of endocrine cells to each other and to other cell types in the human gastric fundus and corpus. Cell Tissue Res. 2019;376(1):37–49.
- Solcia E, Rindi G, Buffa R, Fiocca R, Capella C. Gastric endocrine cells: types, function and growth. Regul. Pept. 2000;93(1-3):31–35.
- Busslinger GA, Weusten BLA, Bogte A, Begthel H, Brosens LAA, Clevers H. Human gastrointestinal epithelia of the esophagus, stomach, and duodenum resolved at single-cell resolution. Cell Rep. 2021;34(10):108819.
- Lee CS, Perreault N, Brestelli JE, Kaestner KH. Neurogenin 3 is essential for the proper specification of gastric enteroendocrine cells and the maintenance of gastric epithelial cell identity. Genes Dev. 2002;16(12):1488–1497.
- Jenny M, Uhl C, Roche C, Duluc I, Guillermin V, Guillemot F, Jensen J, Kedinger M, Gradwohl G. Neurogenin3 is differentially required for endocrine cell fate specification in the intestinal and gastric epithelium. EMBO J. 2002;21(23):6338–6347.
- Kokubu H, Ohtsuka T, Kageyama R. Mash1 is required for neuroendocrine cell development in the glandular stomach. Genes Cells 2008;13(1):41–51.
- Beumer J, Puschhof J, Bauzá-Martinez J, Martínez-Silgado A, Elmentaite R, James KR, Ross A, Hendriks D, Artegiani B, Busslinger GA, Ponsioen B, Andersson-Rolf A, Saftien A, Boot C, Kretzschmar K, Geurts MH, Bar-Ephraim YE, Pleguezuelos-Manzano C, Post Y, Begthel H, van der Linden F, Lopez-Iglesias C, van de Wetering WJ, van der Linden R, Peters PJ, Heck AJR, Goedhart J, Snippert H, Zilbauer M, Teichmann SA, Wu W, Clevers H. High-Resolution mRNA and Secretome Atlas of Human Enteroendocrine Cells. Cell 2020;181(6):1291–1306.e19.
- Gregory RA, Tracy HJ. THE CONSTITUTION AND PROPERTIES OF TWO GASTRINS EXTRACTED FROM HOG ANTRAL MUCOSA. Gut 1964;5:103–114.
- Grossman MI, Robertson CR, Ivy AC. Proof of a hormonal mechanism for gastric secretion; the humoral transmission of the distention stimulus. Am. J. Physiol. 1948;153(1):1–9.
- Engevik AC, Kaji I, Goldenring JR. The Physiology of the Gastric Parietal Cell. Physiol. Rev. 2020;100(2):573–602.
- Liddle RA. Interactions of Gut Endocrine Cells with Epithelium and Neurons. Compr. Physiol. 2018;8(3):1019–1030.
- Gribble FM, Reimann F. Function and mechanisms of enteroendocrine cells and gut hormones in metabolism. Nature Reviews Endocrinology 2019;15(4):226–237.
- Thomas RP, Hellmich MR, Townsend CM Jr, Evers BM. Role of gastrointestinal hormones in the proliferation of normal and neoplastic tissues. Endocr. Rev. 2003;24(5):571–599.
- Akazawa C, Ishibashi M, Shimizu C, Nakanishi S, Kageyama R. A Mammalian Helix-Loop-Helix Factor Structurally Related to the Product of Drosophila Proneural Gene atonal Is a Positive Transcriptional Regulator Expressed in the Developing Nervous System*. J. Biol. Chem. 1995;270(15):8730–8738.
- Jensen J, Pedersen EE, Galante P, Hald J, Heller RS, Ishibashi M, Kageyama R, Guillemot F, Serup P, Madsen OD. Control of endodermal endocrine development by Hes-1. Nat. Genet. 2000;24(1):36–44.
- Shroyer NF, Helmrath MA, Wang VY-C, Antalffy B, Henning SJ, Zoghbi HY. Intestine-specific ablation of mouse atonal homolog 1 (Math1) reveals a role in cellular homeostasis. Gastroenterology 2007;132(7):2478–2488.
- VanDussen KL, Carulli AJ, Keeley TM, Patel SR, Puthoff BJ, Magness ST, Tran IT, Maillard I, Siebel C, Kolterud Å, Grosse AS, Gumucio DL, Ernst SA, Tsai Y-H, Dempsey PJ, Samuelson LC. Notch signaling modulates proliferation and differentiation of intestinal crypt base columnar stem cells. Development 2012;139(3):488–497.
- Mellitzer G, Beucher A, Lobstein V, Michel P, Robine S, Kedinger M, Gradwohl G. Loss of enteroendocrine cells in mice alters lipid absorption and glucose homeostasis and impairs postnatal survival. J. Clin. Invest. 2010;120(5):1708–1721.
- López-Díaz L, Jain RN, Keeley TM, VanDussen KL, Brunkan CS, Gumucio DL, Samuelson LC. Intestinal Neurogenin 3 directs differentiation of a bipotential secretory progenitor to endocrine cell rather than goblet cell fate. Dev. Biol. 2007;309(2):298–305.
- Wang J, Cortina G, Wu SV, Tran R, Cho J-H, Tsai M-J, Bailey TJ, Jamrich M, Ament ME, Treem WR, Hill ID, Vargas JH, Gershman G, Farmer DG, Reyen L, Martín MG. Mutant neurogenin-3 in congenital malabsorptive diarrhea. N. Engl. J. Med. 2006;355(3):270–280.
- Larsson LI, St-Onge L, Hougaard DM, Sosa-Pineda B, Gruss P. Pax 4 and 6 regulate gastrointestinal endocrine cell development. Mech. Dev. 1998;79(1-2):153–159.
- Desai S, Loomis Z, Pugh-Bernard A, Schrunk J, Doyle MJ, Minic A, McCoy E, Sussel L. Nkx2.2 regulates cell fate choice in the enteroendocrine cell lineages of the intestine. Dev. Biol. 2008;313(1):58–66.
- Gierl MS, Karoulias N, Wende H, Strehle M, Birchmeier C. The zinc-finger factor Insm1 (IA-1) is essential for the development of pancreatic beta cells and intestinal endocrine cells. Genes Dev. 2006;20(17):2465–2478.
- Offield MF, Jetton TL, Labosky PA, Ray M, Stein RW, Magnuson MA, Hogan BL, Wright CV. PDX-1 is required for pancreatic outgrowth and differentiation of the rostral duodenum. Development 1996;122(3):983–995.
- Miyatsuka T, Kosaka Y, Kim H, German MS. Neurogenin3 inhibits proliferation in endocrine progenitors by inducing Cdkn1a. Proc. Natl. Acad. Sci. U. S. A. 2011;108(1):185–190.
- Basak O, Beumer J, Wiebrands K, Seno H, van Oudenaarden A, Clevers H. Induced Quiescence of Lgr5+ Stem Cells in Intestinal Organoids Enables Differentiation of Hormone-Producing Enteroendocrine Cells. Cell Stem Cell 2017;20(2):177–190.e4.
- Gehart H, van Es JH, Hamer K, Beumer J, Kretzschmar K, Dekkers JF, Rios A, Clevers H. Identification of Enteroendocrine Regulators by Real-Time Single-Cell Differentiation Mapping. Cell 2019;176(5):1158–1173.e16.
- Gribble FM, Reimann F. Enteroendocrine Cells: Chemosensors in the Intestinal Epithelium. Annu. Rev. Physiol. 2016;78:277–299.
- Roth KA, Gordon JI. Spatial differentiation of the intestinal epithelium: analysis of enteroendocrine cells containing immunoreactive serotonin, secretin, and substance P in normal and transgenic mice. Proc. Natl. Acad. Sci. U. S. A. 1990;87(16):6408–6412.
- Haber AL, Biton M, Rogel N, Herbst RH, Shekhar K, Smillie C, Burgin G, Delorey TM, Howitt MR, Katz Y, Tirosh I, Beyaz S, Dionne D, Zhang M, Raychowdhury R, Garrett WS, Rozenblatt-Rosen O, Shi HN, Yilmaz O, Xavier RJ, Regev A. A single-cell survey of the small intestinal epithelium. Nature 2017;551(7680):333–339.
- Habib AM, Richards P, Cairns LS, Rogers GJ, Bannon CAM, Parker HE, Morley TCE, Yeo GSH, Reimann F, Gribble FM. Overlap of endocrine hormone expression in the mouse intestine revealed by transcriptional profiling and flow cytometry. Endocrinology 2012;153(7):3054–3065.
- Diwakarla S, Fothergill LJ, Fakhry J, Callaghan B, Furness JB. Heterogeneity of enterochromaffin cells within the gastrointestinal tract. Neurogastroenterol. Motil. 2017;29(6). doi:10.1111/nmo.13101.
- Fothergill LJ, Callaghan B, Hunne B, Bravo DM, Furness JB. Costorage of Enteroendocrine Hormones Evaluated at the Cell and Subcellular Levels in Male Mice. Endocrinology 2017;158(7):2113–2123.
- Inokuchi H, Azuma T, Kawai K, Takeuchi Y, Sano Y. Serotonin immunohistochemistry reveals immature EC cells. Histochemistry 1984;80(5):517–518.
- Inokuchi H, Fujimoto S, Hattori T, Kawai K. Tritiated thymidine radioautographic study on the origin and renewal of secretin cells in the rat duodenum. Gastroenterology 1985;89(5):1014–1020.
- Aiken KD, Roth KA. Temporal differentiation and migration of substance P, serotonin, and secretin immunoreactive enteroendocrine cells in the mouse proximal small intestine. Dev. Dyn. 1992;194(4):303–310.
- Grunddal KV, Ratner CF, Svendsen B, Sommer F, Engelstoft MS, Madsen AN, Pedersen J, Nøhr MK, Egerod KL, Nawrocki AR, Kowalski T, Howard AD, Poulsen SS, Offermanns S, Bäckhed F, Holst JJ, Holst B, Schwartz TW. Neurotensin Is Coexpressed, Coreleased, and Acts Together With GLP-1 and PYY in Enteroendocrine Control of Metabolism. Endocrinology 2016;157(1):176–194.
- Beumer J, Artegiani B, Post Y, Reimann F, Gribble F, Nguyen TN, Zeng H, Van den Born M, Van Es JH, Clevers H. Enteroendocrine cells switch hormone expression along the crypt-to-villus BMP signalling gradient. Nat. Cell Biol. 2018;20(8):909–916.
- Sjölund K, Sandén G, Håkanson R, Sundler F. Endocrine cells in human intestine: an immunocytochemical study. Gastroenterology 1983;85(5):1120–1130.
- Cristina ML, Lehy T, Zeitoun P, Dufougeray F. Fine structural classification and comparative distribution of endocrine cells in normal human large intestine. Gastroenterology 1978;75(1):20–28.
- Gunawardene AR, Corfe BM, Staton CA. Classification and functions of enteroendocrine cells of the lower gastrointestinal tract. Int. J. Exp. Pathol. 2011;92(4):219–231.
- Modlin IM, Kidd M, Pfragner R, Eick GN, Champaneria MC. The functional characterization of normal and neoplastic human enterochromaffin cells. J. Clin. Endocrinol. Metab. 2006;91(6):2340–2348.
- Brummelte S, Mc Glanaghy E, Bonnin A, Oberlander TF. Developmental changes in serotonin signaling: Implications for early brain function, behavior and adaptation. Neuroscience 2017;342:212–231.
- Terry N, Margolis KG. Serotonergic Mechanisms Regulating the GI Tract: Experimental Evidence and Therapeutic Relevance. Handb. Exp. Pharmacol. 2017;239:319–342.
- Mawe GM, Hoffman JM. Serotonin signalling in the gut--functions, dysfunctions and therapeutic targets. Nat. Rev. Gastroenterol. Hepatol. 2013;10(8):473–486.
- Bellono NW, Bayrer JR, Leitch DB, Castro J, Zhang C, O’Donnell TA, Brierley SM, Ingraham HA, Julius D. Enterochromaffin Cells Are Gut Chemosensors that Couple to Sensory Neural Pathways. Cell 2017;170(1):185–198.e16.
- Alcaino C, Knutson KR, Treichel AJ, Yildiz G, Strege PR, Linden DR, Li JH, Leiter AB, Szurszewski JH, Farrugia G, Beyder A. A population of gut epithelial enterochromaffin cells is mechanosensitive and requires Piezo2 to convert force into serotonin release. Proc. Natl. Acad. Sci. U. S. A. 2018;115(32):E7632–E7641.
- Lundqvist M, Wilander E. A study of the histopathogenesis of carcinoid tumors of the small intestine and appendix. Cancer 1987;60(2):201–206.
- Moyana TN, Satkunam N. A comparative immunohistochemical study of jejunoileal and appendiceal carcinoids. Implications for histogenesis and pathogenesis. Cancer 1992;70(5):1081–1088.
- Sei Y, Feng J, Zhao X, Wank SA. Role of an active reserve stem cell subset of enteroendocrine cells in intestinal stem cell dynamics and the genesis of small intestinal neuroendocrine tumors. Am. J. Physiol. Gastrointest. Liver Physiol. 2020;319(4):G494–G501.
- Sei Y, Feng J, Samsel L, White A, Zhao X, Yun S, Citrin D, McCoy JP, Sundaresan S, Hayes MM, Merchant JL, Leiter A, Wank SA. Mature enteroendocrine cells contribute to basal and pathological stem cell dynamics in the small intestine. Am. J. Physiol. Gastrointest. Liver Physiol. 2018;315(4):G495–G510.
- Sei Y, Feng J, Zhao X, Forbes J, Tang D, Nagashima K, Hanson J, Quezado MM, Hughes MS, Wank SA. Polyclonal Crypt Genesis and Development of Familial Small Intestinal Neuroendocrine Tumors. Gastroenterology 2016;151(1):140–151.
- Muñoz J, Stange DE, Schepers AG, van de Wetering M, Koo B-K, Itzkovitz S, Volckmann R, Kung KS, Koster J, Radulescu S, Myant K, Versteeg R, Sansom OJ, van Es JH, Barker N, van Oudenaarden A, Mohammed S, Heck AJR, Clevers H. The Lgr5 intestinal stem cell signature: robust expression of proposed quiescent “+4” cell markers. EMBO J. 2012;31(14):3079–3091.
- Beumer J, Clevers H. Regulation and plasticity of intestinal stem cells during homeostasis and regeneration. Development 2016;143(20):3639–3649.
- Worthington JJ, Reimann F, Gribble FM. Enteroendocrine cells-sensory sentinels of the intestinal environment and orchestrators of mucosal immunity. Mucosal Immunol. 2018;11(1):3–20.
- Sciola V, Massironi S, Conte D, Caprioli F, Ferrero S, Ciafardini C, Peracchi M, Bardella MT, Piodi L. Plasma chromogranin a in patients with inflammatory bowel disease. Inflamm. Bowel Dis. 2009;15(6):867–871.
- El-Salhy M, Gundersen D, Hatlebakk JG, Hausken T. Chromogranin A cell density as a diagnostic marker for lymphocytic colitis. Dig. Dis. Sci. 2012;57(12):3154–3159.
- El-Salhy M, Gilja OH. Abnormalities in ileal stem, neurogenin 3, and enteroendocrine cells in patients with irritable bowel syndrome. BMC Gastroenterol. 2017;17(1):90.
- Rioux JD, Xavier RJ, Taylor KD, Silverberg MS, Goyette P, Huett A, Green T, Kuballa P, Barmada MM, Datta LW, Shugart YY, Griffiths AM, Targan SR, Ippoliti AF, Bernard E-J, Mei L, Nicolae DL, Regueiro M, Schumm LP, Steinhart AH, Rotter JI, Duerr RH, Cho JH, Daly MJ, Brant SR. Genome-wide association study identifies new susceptibility loci for Crohn disease and implicates autophagy in disease pathogenesis. Nat. Genet. 2007;39(5):596–604.
- Hernández-Trejo JA, Suárez-Pérez D, Gutiérrez-Martínez IZ, Fernandez-Vargas OE, Serrano C, Candelario-Martínez AA, Meraz-Ríos MA, Citalán-Madrid AF, Hernández-Ruíz M, Reyes-Maldonado E, Valle-Rios R, Feintuch-Unger JH, Schnoor M, Villegas-Sepúlveda N, Medina-Contreras O, Nava P. The pro-inflammatory cytokines IFNγ/TNFα increase chromogranin A-positive neuroendocrine cells in the colonic epithelium. Biochem. J 2016;473(21):3805–3818.
- Manocha M, Shajib MS, Rahman MM, Wang H, Rengasamy P, Bogunovic M, Jordana M, Mayer L, Khan WI. IL-13-mediated immunological control of enterochromaffin cell hyperplasia and serotonin production in the gut. Mucosal Immunol. 2013;6(1):146–155.
- Worthington JJ. The intestinal immunoendocrine axis: novel cross-talk between enteroendocrine cells and the immune system during infection and inflammatory disease. Biochem. Soc. Trans. 2015;43(4):727–733.
- Friedrich M, Diegelmann J, Schauber J, Auernhammer CJ, Brand S. Intestinal neuroendocrine cells and goblet cells are mediators of IL-17A-amplified epithelial IL-17C production in human inflammatory bowel disease. Mucosal Immunol. 2015;8(4):943–958.
- Bogunovic M, Davé SH, Tilstra JS, Chang DTW, Harpaz N, Xiong H, Mayer LF, Plevy SE. Enteroendocrine cells express functional Toll-like receptors. Am. J. Physiol. Gastrointest. Liver Physiol. 2007;292(6):G1770–83.
- Rindi G, Solcia E. Endocrine hyperplasia and dysplasia in the pathogenesis of gastrointestinal and pancreatic endocrine tumors. Gastroenterol. Clin. North Am. 2007;36(4):851–65, vi.
- Sheng W, Malagola E, Nienhüser H, Zhang Z, Kim W, Zamechek L, Sepulveda A, Hata M, Hayakawa Y, Zhao C-M, Chen D, Wang TC. Hypergastrinemia Expands Gastric ECL Cells Through CCK2R+ Progenitor Cells via ERK Activation. Cell Mol Gastroenterol Hepatol 2020;10(2):434–449.e1.
- van Es JH, Sato T, van de Wetering M, Lyubimova A, Yee Nee AN, Gregorieff A, Sasaki N, Zeinstra L, van den Born M, Korving J, Martens ACM, Barker N, van Oudenaarden A, Clevers H. Dll1+ secretory progenitor cells revert to stem cells upon crypt damage. Nat. Cell Biol. 2012;14(10):1099–1104.
- Yan KS, Chia LA, Li X, Ootani A, Su J, Lee JY, Su N, Luo Y, Heilshorn SC, Amieva MR, Sangiorgi E, Capecchi MR, Kuo CJ. The intestinal stem cell markers Bmi1 and Lgr5 identify two functionally distinct populations. Proc. Natl. Acad. Sci. U. S. A. 2012;109(2):466–471.
- Buczacki SJA, Zecchini HI, Nicholson AM, Russell R, Vermeulen L, Kemp R, Winton DJ. Intestinal label-retaining cells are secretory precursors expressing Lgr5. Nature 2013;495(7439):65–69.
- Tetteh PW, Basak O, Farin HF, Wiebrands K, Kretzschmar K, Begthel H, van den Born M, Korving J, de Sauvage F, van Es JH, van Oudenaarden A, Clevers H. Replacement of Lost Lgr5-Positive Stem Cells through Plasticity of Their Enterocyte-Lineage Daughters. Cell Stem Cell 2016;18(2):203–213.
- Yan KS, Gevaert O, Zheng GXY, Anchang B, Probert CS, Larkin KA, Davies PS, Cheng Z-F, Kaddis JS, Han A, Roelf K, Calderon RI, Cynn E, Hu X, Mandleywala K, Wilhelmy J, Grimes SM, Corney DC, Boutet SC, Terry JM, Belgrader P, Ziraldo SB, Mikkelsen TS, Wang F, von Furstenberg RJ, Smith NR, Chandrakesan P, May R, Chrissy MAS, Jain R, Cartwright CA, Niland JC, Hong Y-K, Carrington J, Breault DT, Epstein J, Houchen CW, Lynch JP, Martin MG, Plevritis SK, Curtis C, Ji HP, Li L, Henning SJ, Wong MH, Kuo CJ. Intestinal Enteroendocrine Lineage Cells Possess Homeostatic and Injury-Inducible Stem Cell Activity. Cell Stem Cell 2017;21(1):78–90.e6.
- van Es JH, Wiebrands K, López-Iglesias C, van de Wetering M, Zeinstra L, van den Born M, Korving J, Sasaki N, Peters PJ, van Oudenaarden A, Clevers H. Enteroendocrine and tuft cells support Lgr5 stem cells on Paneth cell depletion. Proc. Natl. Acad. Sci. U. S. A. 2019. doi:10.1073/pnas.1801888117.
- Röder PV, Wu B, Liu Y, Han W. Pancreatic regulation of glucose homeostasis. Experimental & molecular medicine 2016;48(3):e219.
- Deltour L, Leduque P, Paldi A, Ripoche MA, Dubois P, Jami J. Polyclonal origin of pancreatic islets in aggregation mouse chimaeras. Development 1991;112(4):1115–1121.
- Gradwohl G, Dierich A, LeMeur M, Guillemot F. neurogenin3 is required for the development of the four endocrine cell lineages of the pancreas. Proc. Natl. Acad. Sci. U. S. A. 2000;97(4):1607–1611.
- McGrath PS, Watson CL, Ingram C, Helmrath MA, Wells JM. The Basic Helix-Loop-Helix Transcription Factor NEUROG3 Is Required for Development of the Human Endocrine Pancreas. Diabetes 2015;64(7):2497–2505.
- Rukstalis JM, Habener JF. Neurogenin3: a master regulator of pancreatic islet differentiation and regeneration. Islets 2009;1(3):177–184.
- Villasenor A, Chong DC, Cleaver O. Biphasic Ngn3 expression in the developing pancreas. Dev. Dyn. 2008;237(11):3270–3279.
- Cirulli V, Crisa L, Beattie GM, Mally MI, Lopez AD, Fannon A, Ptasznik A, Inverardi L, Ricordi C, Deerinck T, Ellisman M, Reisfeld RA, Hayek A. KSA Antigen Ep-CAM Mediates Cell–Cell Adhesion of Pancreatic Epithelial Cells: Morphoregulatory Roles in Pancreatic Islet Development. J. Cell Biol. 1998;140(6):1519–1534.
- Cirulli V, Baetens D, Rutishauser U, Halban PA, Orci L, Rouiller DG. Expression of neural cell adhesion molecule (N-CAM) in rat islets and its role in islet cell type segregation. J. Cell Sci. 1994;107 ( Pt 6):1429–1436.
- Pan FC, Brissova M. Pancreas development in humans. Curr. Opin. Endocrinol. Diabetes Obes. 2014;21(2):77–82.
- Jennings RE, Berry AA, Kirkwood-Wilson R, Roberts NA, Hearn T, Salisbury RJ, Blaylock J, Piper Hanley K, Hanley NA. Development of the human pancreas from foregut to endocrine commitment. Diabetes 2013;62(10):3514–3522.
- Steiner DJ, Kim A, Miller K, Hara M. Pancreatic islet plasticity: interspecies comparison of islet architecture and composition. Islets 2010;2(3):135–145.
- Cabrera O, Berman DM, Kenyon NS, Ricordi C, Berggren P-O, Caicedo A. The unique cytoarchitecture of human pancreatic islets has implications for islet cell function. Proc. Natl. Acad. Sci. U. S. A. 2006;103(7):2334–2339.
- Brissova M, Fowler MJ, Nicholson WE, Chu A, Hirshberg B, Harlan DM, Powers AC. Assessment of human pancreatic islet architecture and composition by laser scanning confocal microscopy. J. Histochem. Cytochem. 2005;53(9):1087–1097.
- Hædersdal S, Lund A, Knop FK, Vilsbøll T. The Role of Glucagon in the Pathophysiology and Treatment of Type 2 Diabetes. Mayo Clin. Proc. 2018;93(2):217–239.
- Hancock AS, Du A, Liu J, Miller M, May CL. Glucagon deficiency reduces hepatic glucose production and improves glucose tolerance in adult mice. Mol. Endocrinol. 2010;24(8):1605–1614.
- Collombat P, Hecksher-Sørensen J, Krull J, Berger J, Riedel D, Herrera PL, Serup P, Mansouri A. Embryonic endocrine pancreas and mature beta cells acquire alpha and PP cell phenotypes upon Arx misexpression. J. Clin. Invest. 2007;117(4):961–970.
- Artner I, Blanchi B, Raum JC, Guo M, Kaneko T, Cordes S, Sieweke M, Stein R. MafB is required for islet beta cell maturation. Proc. Natl. Acad. Sci. U. S. A. 2007;104(10):3853–3858.
- Katoh MC, Jung Y, Ugboma CM, Shimbo M, Kuno A, Basha WA, Kudo T, Oishi H, Takahashi S. MafB Is Critical for Glucagon Production and Secretion in Mouse Pancreatic α Cells In Vivo. Mol. Cell. Biol. 2018;38(8). doi:10.1128/MCB.00504-17.
- Jung S-H, Jung C-H, Reaven GM, Kim SH. Adapting to insulin resistance in obesity: role of insulin secretion and clearance. Diabetologia 2018;61(3):681–687.
- Iozzo P, Hallsten K, Oikonen V, Virtanen KA, Kemppainen J, Solin O, Ferrannini E, Knuuti J, Nuutila P. Insulin-mediated hepatic glucose uptake is impaired in type 2 diabetes: evidence for a relationship with glycemic control. J. Clin. Endocrinol. Metab. 2003;88(5):2055–2060.
- Chadt A, Al-Hasani H. Glucose transporters in adipose tissue, liver, and skeletal muscle in metabolic health and disease. Pflugers Arch. 2020;472(9):1273–1298.
- Petersen MC, Shulman GI. Mechanisms of Insulin Action and Insulin Resistance. Physiol. Rev. 2018;98(4):2133–2223.
- Sussel L, Kalamaras J, Hartigan-O’Connor DJ, Meneses JJ, Pedersen RA, Rubenstein JL, German MS. Mice lacking the homeodomain transcription factor Nkx2.2 have diabetes due to arrested differentiation of pancreatic beta cells. Development 1998;125(12):2213–2221.
- Sander M, Sussel L, Conners J, Scheel D, Kalamaras J, Dela Cruz F, Schwitzgebel V, Hayes-Jordan A, German M. Homeobox gene Nkx6.1 lies downstream of Nkx2.2 in the major pathway of beta-cell formation in the pancreas. Development 2000;127(24):5533–5540.
- Gasa R, Mrejen C, Lynn FC, Skewes-Cox P, Sanchez L, Yang KY, Lin C-H, Gomis R, German MS. Induction of pancreatic islet cell differentiation by the neurogenin-neuroD cascade. Differentiation 2008;76(4):381–391.
- Naya FJ, Huang HP, Qiu Y, Mutoh H, DeMayo FJ, Leiter AB, Tsai MJ. Diabetes, defective pancreatic morphogenesis, and abnormal enteroendocrine differentiation in BETA2/neuroD-deficient mice. Genes Dev. 1997;11(18):2323–2334.
- Russell R, Carnese PP, Hennings TG, Walker EM, Russ HA, Liu JS, Giacometti S, Stein R, Hebrok M. Loss of the transcription factor MAFB limits β-cell derivation from human PSCs. Nat. Commun. 2020;11(1):2742.
- Zhang C, Moriguchi T, Kajihara M, Esaki R, Harada A, Shimohata H, Oishi H, Hamada M, Morito N, Hasegawa K, Kudo T, Engel JD, Yamamoto M, Takahashi S. MafA is a key regulator of glucose-stimulated insulin secretion. Mol. Cell. Biol. 2005;25(12):4969–4976.
- Arrojo E Drigo R, Jacob S, García-Prieto CF, Zheng X, Fukuda M, Nhu HTT, Stelmashenko O, Peçanha FLM, Rodriguez-Diaz R, Bushong E, Deerinck T, Phan S, Ali Y, Leibiger I, Chua M, Boudier T, Song S-H, Graf M, Augustine GJ, Ellisman MH, Berggren P-O. Structural basis for delta cell paracrine regulation in pancreatic islets. Nat. Commun. 2019;10(1):3700.
- Moss CE, Marsh WJ, Parker HE, Ogunnowo-Bada E, Riches CH, Habib AM, Evans ML, Gribble FM, Reimann F. Somatostatin receptor 5 and cannabinoid receptor 1 activation inhibit secretion of glucose-dependent insulinotropic polypeptide from intestinal K cells in rodents. Diabetologia 2012;55(11):3094–3103.
- Vergari E, Knudsen JG, Ramracheya R, Salehi A, Zhang Q, Adam J, Asterholm IW, Benrick A, Briant LJB, Chibalina MV, Gribble FM, Hamilton A, Hastoy B, Reimann F, Rorsman NJG, Spiliotis II, Tarasov A, Wu Y, Ashcroft FM, Rorsman P. Insulin inhibits glucagon release by SGLT2-induced stimulation of somatostatin secretion. Nat. Commun. 2019;10(1):139.
- Gong A-Y, Tietz PS, Muff MA, Splinter PL, Huebert RC, Strowski MZ, Chen X-M, LaRusso NF. Somatostatin stimulates ductal bile absorption and inhibits ductal bile secretion in mice via SSTR2 on cholangiocytes. Am. J. Physiol. Cell Physiol. 2003;284(5):C1205–14.
- Zhang J, McKenna LB, Bogue CW, Kaestner KH. The diabetes gene Hhex maintains δ-cell differentiation and islet function. Genes Dev. 2014;28(8):829–834.
- Billings LK, Florez JC. The genetics of type 2 diabetes: what have we learned from GWAS? Ann. N. Y. Acad. Sci. 2010;1212:59–77.
- Sakata N, Yoshimatsu G, Kodama S. Development and Characteristics of Pancreatic Epsilon Cells. Int. J. Mol. Sci. 2019;20(8). doi:10.3390/ijms20081867.
- Kojima M. The discovery of ghrelin--a personal memory. Regul. Pept. 2008;145(1-3):2–6.
- Koutouratsas T, Kalli T, Karamanolis G, Gazouli M. Contribution of ghrelin to functional gastrointestinal disorders’ pathogenesis. World J. Gastroenterol. 2019;25(5):539–551.
- Pradhan G, Samson SL, Sun Y. Ghrelin: much more than a hunger hormone. Curr. Opin. Clin. Nutr. Metab. Care 2013;16(6):619–624.
- Broglio F, Gottero C, Prodam F, Destefanis S, Gauna C, Me E, Riganti F, Vivenza D, Rapa A, Martina V, Arvat E, Bona G, van der Lely AJ, Ghigo E. Ghrelin secretion is inhibited by glucose load and insulin-induced hypoglycaemia but unaffected by glucagon and arginine in humans. Clinical Endocrinology 2004;61(4):503–509.
- Arafat MA, Otto B, Rochlitz H, Tschöp M, Bähr V, Möhlig M, Diederich S, Spranger J, Pfeiffer AFH. Glucagon inhibits ghrelin secretion in humans. Eur. J. Endocrinol. 2005;153(3):397–402.
- Banasch M, Bulut K, Hagemann D, Schrader H, Holst JJ, Schmidt WE, Meier JJ. Glucagon-like peptide 2 inhibits ghrelin secretion in humans. Regul. Pept. 2006;137(3):173–178.
- DiGruccio MR, Mawla AM, Donaldson CJ, Noguchi GM, Vaughan J, Cowing-Zitron C, van der Meulen T, Huising MO. Comprehensive alpha, beta and delta cell transcriptomes reveal that ghrelin selectively activates delta cells and promotes somatostatin release from pancreatic islets. Mol Metab 2016;5(7):449–458.
- Adriaenssens AE, Svendsen B, Lam BYH, Yeo GSH, Holst JJ, Reimann F, Gribble FM. Transcriptomic profiling of pancreatic alpha, beta and delta cell populations identifies delta cells as a principal target for ghrelin in mouse islets. Diabetologia 2016;59(10):2156–2165.
- Heller RS, Jenny M, Collombat P, Mansouri A, Tomasetto C, Madsen OD, Mellitzer G, Gradwohl G, Serup P. Genetic determinants of pancreatic epsilon-cell development. Dev. Biol. 2005;286(1):217–224.
- Hart AW, Mella S, Mendrychowski J, van Heyningen V, Kleinjan DA. The developmental regulator Pax6 is essential for maintenance of islet cell function in the adult mouse pancreas. PLoS One 2013;8(1):e54173.
- Ahmad Z, Rafeeq M, Collombat P, Mansouri A. Pax6 Inactivation in the Adult Pancreas Reveals Ghrelin as Endocrine Cell Maturation Marker. PLoS One 2015;10(12):e0144597.
- Brereton MF, Vergari E, Zhang Q, Clark A. Alpha-, Delta- and PP-cells: Are They the Architectural Cornerstones of Islet Structure and Co-ordination? J. Histochem. Cytochem. 2015;63(8):575–591.
- Batterham RL, Le Roux CW, Cohen MA, Park AJ, Ellis SM, Patterson M, Frost GS, Ghatei MA, Bloom SR. Pancreatic polypeptide reduces appetite and food intake in humans. J. Clin. Endocrinol. Metab. 2003;88(8):3989–3992.
- Ueno N, Inui A, Iwamoto M, Kaga T, Asakawa A, Okita M, Fujimiya M, Nakajima Y, Ohmoto Y, Ohnaka M, Nakaya Y, Miyazaki JI, Kasuga M. Decreased food intake and body weight in pancreatic polypeptide-overexpressing mice. Gastroenterology 1999;117(6):1427–1432.
- Batterham RL, Cohen MA, Ellis SM, Le Roux CW, Withers DJ, Frost GS, Ghatei MA, Bloom SR. Inhibition of Food Intake in Obese Subjects by Peptide YY3–36. N. Engl. J. Med. 2003;349(10):941–948.
- Aragón F, Karaca M, Novials A, Maldonado R, Maechler P, Rubí B. Pancreatic polypeptide regulates glucagon release through PPYR1 receptors expressed in mouse and human alpha-cells. Biochim. Biophys. Acta 2015;1850(2):343–351.
- Kim W, Fiori JL, Shin YK, Okun E, Kim JS, Rapp PR, Egan JM. Pancreatic polypeptide inhibits somatostatin secretion. FEBS Lett. 2014;588(17):3233–3239.
- Hyland NP, Pittman QJ, Sharkey KA. Peptide YY containing enteroendocrine cells and peripheral tissue sensitivity to PYY and PYY(3-36) are maintained in diet-induced obese and diet-resistant rats. Peptides 2007;28(6):1185–1190.
- Berrocal T, Luque AA, Pinilla I, Lassaletta L. Pancreatic regeneration after near-total pancreatectomy in children with nesidioblastosis. Pediatr. Radiol. 2005;35(11):1066–1070.
- Lehv M, Fitzgerald PJ. Pancreatic acinar cell regeneration. IV. Regeneration after resection. Am. J. Pathol. 1968;53(4):513–535.
- Watanabe H, Saito H, Rychahou PG, Uchida T, Evers BM. Aging is associated with decreased pancreatic acinar cell regeneration and phosphatidylinositol 3-kinase/Akt activation. Gastroenterology 2005;128(5):1391–1404.
- Menge BA, Tannapfel A, Belyaev O, Drescher R, Müller C, Uhl W, Schmidt WE, Meier JJ. Partial pancreatectomy in adult humans does not provoke beta-cell regeneration. Diabetes 2008;57(1):142–149.
- Dor Y, Brown J, Martinez OI, Melton DA. Adult pancreatic beta-cells are formed by self-duplication rather than stem-cell differentiation. Nature 2004;429(6987):41–46.
- Teta M, Rankin MM, Long SY, Stein GM, Kushner JA. Growth and Regeneration of Adult β Cells Does Not Involve Specialized Progenitors. Dev. Cell 2007;12(5):817–826.
- Gu D, Sarvetnick N. Epithelial cell proliferation and islet neogenesis in IFN-g transgenic mice. Development 1993;118(1):33–46.
- Wang RN, Klöppel G, Bouwens L. Duct- to islet-cell differentiation and islet growth in the pancreas of duct-ligated adult rats. Diabetologia 1995;38(12):1405–1411.
- Bonner-Weir S, Baxter LA, Schuppin GT, Smith FE. A Second Pathway for Regeneration of Adult Exocrine and Endocrine Pancreas A Possible Recapitulation of Embryonic Development.; 1993:1715–1735.
- Xu X, D’Hoker J, Stangé G, Bonné S, De Leu N, Xiao X, Van De Casteele M, Mellitzer G, Ling Z, Pipeleers D, Bouwens L, Scharfmann R, Gradwohl G, Heimberg H. β Cells Can Be Generated from Endogenous Progenitors in Injured Adult Mouse Pancreas. Cell 2008;132(2):197–207.
- Inada A, Nienaber C, Katsuta H, Fujitani Y, Levine J, Morita R, Sharma A, Bonner-Weir S. Carbonic anhydrase II-positive pancreatic cells are progenitors for both endocrine and exocrine pancreas after birth.; 2008. Available at: www.pnas.org/cgi/content/full/.
- Thorel F, Népote V, Avril I, Kohno K, Desgraz R, Chera S, Herrera PL. Conversion of adult pancreatic α-cells to β-cells after extreme β-cell loss. Nature 2010;464(7292):1149–1154.
- Heit JJ, Karnik SK, Kim SK. Intrinsic regulators of pancreatic beta-cell proliferation. Annu. Rev. Cell Dev. Biol. 2006;22:311–338.
- Ackermann AM, Gannon M. Molecular regulation of pancreatic beta-cell mass development, maintenance, and expansion. J. Mol. Endocrinol. 2007;38(1-2):193–206.
- Ouyang D, Dhall D, Yu R. Pathologic pancreatic endocrine cell hyperplasia. World J. Gastroenterol. 2011;17(2):137–143.
- Rahier J, Wallon J, Henquin JC. Cell populations in the endocrine pancreas of human neonates and infants. Diabetologia 1981;20(5):540–546.
- Klöppel G, Reinecke-Lüthge A, Koschoreck F. Focal and Diffuse Beta Cell Changes in Persistent Hyperinsulinemic Hypoglycemia of Infancy. Endocr. Pathol. 1999;10(4):299–304.
- Rahier J, Guiot Y, Sempoux C. Persistent hyperinsulinaemic hypoglycaemia of infancy: a heterogeneous syndrome unrelated to nesidioblastosis. Arch. Dis. Child. Fetal Neonatal Ed. 2000;82(2):F108–12.
- Thompson GB, Service FJ, Andrews JC, Lloyd RV, Natt N, van Heerden JA, Grant CS. Noninsulinoma pancreatogenous hypoglycemia syndrome: an update in 10 surgically treated patients. Surgery 2000;128(6):937–44;discussion 944–5.
- Won JGS, Tseng H-S, Yang A-H, Tang K-T, Jap T-S, Lee CH, Lin H-D, Burcus N, Pittenger G, Vinik A. Clinical features and morphological characterization of 10 patients with noninsulinoma pancreatogenous hypoglycaemia syndrome (NIPHS). Clin. Endocrinol.2006;65(5):566–578.
- Jack MM, Walker RM, Thomsett MJ, Cotterill AM, Bell JR. Histologic findings in persistent hyperinsulinemic hypoglycemia of infancy: Australian experience. Pediatr. Dev. Pathol. 2000;3(6):532–547.
- Maguire DJ, Bruley DF, Harrison DK, eds. Oxygen Transport to Tissue XXVIII. Springer, Boston, MA; 2008.
- Anlauf M, Wieben D, Perren A, Sipos B, Komminoth P, Raffel A, Kruse ML, Fottner C, Knoefel WT, Mönig H, Heitz PU, Klöppel G. Persistent hyperinsulinemic hypoglycemia in 15 adults with diffuse nesidioblastosis: diagnostic criteria, incidence, and characterization of beta-cell changes. Am. J. Surg. Pathol. 2005;29(4):524–533.
- Service FJ, Natt N, Thompson GB, Grant CS, van Heerden JA, Andrews JC, Lorenz E, Terzic A, Lloyd RV. Noninsulinoma pancreatogenous hypoglycemia: a novel syndrome of hyperinsulinemic hypoglycemia in adults independent of mutations in Kir6.2 and SUR1 genes. J. Clin. Endocrinol. Metab. 1999;84(5):1582–1589.
- Sipos B, Sperveslage J, Anlauf M, Hoffmeister M, Henopp T, Buch S, Hampe J, Weber A, Hammel P, Couvelard A, Höbling W, Lieb W, Boehm BO, Klöppel G. Glucagon cell hyperplasia and neoplasia with and without glucagon receptor mutations. J. Clin. Endocrinol. Metab. 2015;100(5):E783–8.
- Zhou C, Dhall D, Nissen NN, Chen C-R, Yu R. Homozygous P86S mutation of the human glucagon receptor is associated with hyperglucagonemia, alpha cell hyperplasia, and islet cell tumor. Pancreas 2009;38(8):941–946.
- Henopp T, Anlauf M, Schmitt A, Schlenger R, Zalatnai A, Couvelard A, Ruszniewski P, Schaps K-P, Jonkers YMH, Speel E-JM, Pellegata NS, Heitz PU, Komminoth P, Perren A, Klöppel G. Glucagon cell adenomatosis: a newly recognized disease of the endocrine pancreas. J. Clin. Endocrinol. Metab. 2009;94(1):213–217.
- Dean ED. A Primary Role for α-Cells as Amino Acid Sensors. Diabetes 2020;69(4):542–549.
- Albazaz R, Da Costa PE, Verbeke CS. Pancreatic polypeptide cell hyperplasia of the pancreas. J. Clin. Pathol. 2006;59(10):1087–1090.
- Martella EM, Ferraro G, Azzoni C, Marignani M, Bordi C. Pancreatic-polypeptide cell hyperplasia associated with pancreatic or duodenal gastrinomas. Hum. Pathol. 1997;28(2):149–153.
- Tomita T, Kimmel JR, Friesen SR, Mantz FA Jr. Pancreatic polypeptide cell hyperplasia with and without watery diarrhea syndrome. J. Surg. Oncol. 1980;14(1):11–20.
- Lee MR, Harris C, Baeg KJ, Aronson A, Wisnivesky JP, Kim MK. Incidence Trends of Gastroenteropancreatic Neuroendocrine Tumors in the United States. Clin. Gastroenterol. Hepatol. 2019;17(11):2212–2217.e1.
- Dasari A, Shen C, Halperin D, Zhao B, Zhou S, Xu Y, Shih T, Yao JC. Trends in the Incidence, Prevalence, and Survival Outcomes in Patients With Neuroendocrine Tumors in the United States. JAMA Oncol 2017;3(10):1335–1342.
- Sorbye H, Strosberg J, Baudin E, Klimstra DS, Yao JC. Gastroenteropancreatic high-grade neuroendocrine carcinoma. Cancer 2014;120(18):2814–2823.
- Nagtegaal ID, Odze RD, Klimstra D, Paradis V, Rugge M, Schirmacher P, Washington KM, Carneiro F, Cree IA, WHO Classification of Tumours Editorial Board. The 2019 WHO classification of tumours of the digestive system. Histopathology 2020;76(2):182–188.
- Konukiewitz B, Jesinghaus M, Steiger K, Schlitter AM, Kasajima A, Sipos B, Zamboni G, Weichert W, Pfarr N, Klöppel G. Pancreatic neuroendocrine carcinomas reveal a closer relationship to ductal adenocarcinomas than to neuroendocrine tumors G3. Hum. Pathol. 2018;77:70–79.
- Neychev V, Steinberg SM, Cottle-Delisle C, Merkel R. Mutation-targeted therapy with sunitinib or everolimus in patients with advanced low-grade or intermediate-grade neuroendocrine tumours of the gastrointestinal tract …. BMJ Open 2015. Available at: https://bmjopen.bmj.com/content/5/5/e008248.short.
- Nishikura K, Watanabe H, Iwafuchi M, Fujiwara T, Kojima K, Ajioka Y. Carcinogenesis of gastric endocrine cell carcinoma: analysis of histopathology and p53 gene alteration. Gastric Cancer 2003;6(4):203–209.
- Makuuchi R, Terashima M, Kusuhara M, Nakajima T, Serizawa M, Hatakeyama K, Ohshima K, Urakami K, Yamaguchi K. Comprehensive analysis of gene mutation and expression profiles in neuroendocrine carcinomas of the stomach. Biomed. Res. 2017;38(1):19–27.
- Takizawa N, Ohishi Y, Hirahashi M, Takahashi S, Nakamura K, Tanaka M, Oki E, Takayanagi R, Oda Y. Molecular characteristics of colorectal neuroendocrine carcinoma; similarities with adenocarcinoma rather than neuroendocrine tumor. Hum. Pathol. 2015;46(12):1890–1900.
- Mafficini A, Scarpa A. Genetics and Epigenetics of Gastroenteropancreatic Neuroendocrine Neoplasms. Endocr. Rev. 2019;40(2):506–536.
- Nassar H, Albores-Saavedra J, Klimstra DS. High-grade neuroendocrine carcinoma of the ampulla of vater: a clinicopathologic and immunohistochemical analysis of 14 cases. Am. J. Surg. Pathol. 2005;29(5):588–594.
- Pizzi S, Azzoni C, Bassi D, Bottarelli L, Milione M, Bordi C. Genetic alterations in poorly differentiated endocrine carcinomas of the gastrointestinal tract. Cancer 2003;98(6):1273–1282.
- Sahan EK, Erdogan N, Ulusoy I, Samet E, Igdem AA, Gonullu D. P53, KI-67, CD117 expression in gastrointestinal and pancreatic neuroendocrine tumours and evaluation of their correlation with clinicopathological and prognostic parameters. The Turkish Journal of Gastroenterology 2015;26(2):104–111.
- Woischke C, Schaaf CW, Yang H-M, Vieth M, Veits L, Geddert H, Märkl B, Stömmer P, Schaeffer DF, Frölich M, Blum H, Vosberg S, Greif PA, Jung A, Kirchner T, Horst D. In-depth mutational analyses of colorectal neuroendocrine carcinomas with adenoma or adenocarcinoma components. Mod. Pathol. 2017;30(1):95–103.
- Puccini A, Poorman K, Salem ME, Soldato D, Seeber A, Goldberg RM, Shields AF, Xiu J, Battaglin F, Berger MD, Tokunaga R, Naseem M, Barzi A, Iqbal S, Zhang W, Soni S, Hwang JJ, Philip PA, Sciallero S, Korn WM, Marshall JL, Lenz H-J. Comprehensive Genomic Profiling of Gastroenteropancreatic Neuroendocrine Neoplasms (GEP-NENs). Clin. Cancer Res. 2020;26(22):5943–5951.
- Detjen K, Hammerich L, Özdirik B, Demir M, Wiedenmann B, Tacke F, Jann H, Roderburg C. Models of Gastroenteropancreatic Neuroendocrine Neoplasms: Current Status and Future Directions. Neuroendocrinology 2021;111(3):217–236.
- Glenn ST, Jones CA, Sexton S, LeVea CM, Caraker SM, Hajduczok G, Gross KW. Conditional deletion of p53 and Rb in the renin-expressing compartment of the pancreas leads to a highly penetrant metastatic pancreatic neuroendocrine carcinoma. Oncogene 2014;33(50):5706–5715.
- Choe J, Kim KW, Kim HJ, Kim DW, Kim KP, Hong S-M, Ryu J-S, Tirumani SH, Krajewski K, Ramaiya N. What Is New in the 2017 World Health Organization Classification and 8th American Joint Committee on Cancer Staging System for Pancreatic Neuroendocrine Neoplasms? Korean J. Radiol. 2019;20(1):5–17.
- Hunter KE, Quick ML, Sadanandam A, Hanahan D, Joyce JA. Identification and characterization of poorly differentiated invasive carcinomas in a mouse model of pancreatic neuroendocrine tumorigenesis. PLoS One 2013;8(5):e64472.
- Kawasaki K, Toshimitsu K, Matano M, Fujita M, Fujii M, Togasaki K, Ebisudani T, Shimokawa M, Takano A, Takahashi S, Ohta Y, Nanki K, Igarashi R, Ishimaru K, Ishida H, Sukawa Y, Sugimoto S, Saito Y, Maejima K, Sasagawa S, Lee H, Kim H-G, Ha K, Hamamoto J, Fukunaga K, Maekawa A, Tanabe M, Ishihara S, Hamamoto Y, Yasuda H, Sekine S, Kudo A, Kitagawa Y, Kanai T, Nakagawa H, Sato T. An Organoid Biobank of Neuroendocrine Neoplasms Enables Genotype-Phenotype Mapping. Cell 2020;183(5):1420–1435.e21.
- Dijkstra KK, van den Berg JG, Weeber F, van de Haar J, Velds A, Kaing S, Peters DDGC, Eskens FALM, de Groot D-JA, Tesselaar MET, Voest EE. Patient-Derived Organoid Models of Human Neuroendocrine Carcinoma. Front. Endocrinol.2021;12:627819.
- Sachs N, Clevers H. Organoid cultures for the analysis of cancer phenotypes. Curr. Opin. Genet. Dev. 2014;24:68–73.
- Mitry E, Baudin E, Ducreux M, Sabourin JC, Rufié P, Aparicio T, Aparicio T, Lasser P, Elias D, Duvillard P, Schlumberger M, Rougier P. Treatment of poorly differentiated neuroendocrine tumours with etoposide and cisplatin. Br. J. Cancer 1999;81(8):1351–1355.
- Sorbye H, Baudin E, Borbath I, Caplin M, Chen J, Cwikla JB, Frilling A, Grossman A, Kaltsas G, Scarpa A, Welin S, Garcia-Carbonero R, ENETS 2016 Munich Advisory Board Participants. Unmet Needs in High-Grade Gastroenteropancreatic Neuroendocrine Neoplasms (WHO G3). Neuroendocrinology 2019;108(1):54–62.
- Kawasaki K, Fujii M, Sato T. Gastroenteropancreatic neuroendocrine neoplasms: genes, therapies and models. Dis. Model. Mech. 2018;11(2). doi:10.1242/dmm.029595.
- McNamara MG, Scoazec J-Y, Walter T. Extrapulmonary poorly differentiated NECs, including molecular and immune aspects. Endocr. Relat. Cancer 2020;27(7):R219–R238.
- Cives M, Strosberg JR. Gastroenteropancreatic Neuroendocrine Tumors. CA Cancer J. Clin. 2018;68(6):471–487.
- Das S, Dasari A. Epidemiology, Incidence, and Prevalence of Neuroendocrine Neoplasms: Are There Global Differences? Curr. Oncol. Rep. 2021;23(4):43.
- Mastracci L, Rindi G, Grillo F, Solcia E, Campora M, Fassan M, Parente P, Vanoli A, La Rosa S. Neuroendocrine neoplasms of the esophagus and stomach. Pathologica 2021;113(1):5–11.
- Tan H. Advances in the diagnosis and treatment of gastric neuroendocrine neoplasms. Transl Gastroenterol Hepatol 2016;1:87.
- Li T-T, Qiu F, Qian ZR, Wan J, Qi X-K, Wu B-Y. Classification, clinicopathologic features and treatment of gastric neuroendocrine tumors. World J. Gastroenterol. 2014;20(1):118–125.
- Modlin IM, Lye KD, Kidd M. A 5-decade analysis of 13,715 carcinoid tumors. Cancer 2003;97(4):934–959.
- Maggard MA, O’Connell JB, Ko CY. Updated population-based review of carcinoid tumors. Ann. Surg. 2004;240(1):117–122.
- Zhang Z, Mäkinen N, Kasai Y, Kim GE, Diosdado B, Nakakura E, Meyerson M. Patterns of chromosome 18 loss of heterozygosity in multifocal ileal neuroendocrine tumors. Genes Chromosomes Cancer 2020;59(9):535–539.
- Norlén O, Stålberg P, Öberg K, Eriksson J, Hedberg J, Hessman O, Janson ET, Hellman P, Åkerström G. Long-term results of surgery for small intestinal neuroendocrine tumors at a tertiary referral center. World J. Surg. 2012;36(6):1419–1431.
- Fanciulli G, Ruggeri RM, Grossrubatscher E, Calzo FL, Wood TD, Faggiano A, Isidori A, Colao A, NIKE. Serotonin pathway in carcinoid syndrome: Clinical, diagnostic, prognostic and therapeutic implications. Rev. Endocr. Metab. Disord. 2020;21(4):599–612.
- Robertson JI. Carcinoid syndrome and serotonin: therapeutic effects of ketanserin. Cardiovasc. Drugs Ther. 1990;4 Suppl 1:53–58.
- Pandit S, Annamaraju P, Bhusal K. Carcinoid Syndrome. In: StatPearls. Treasure Island (FL): StatPearls Publishing; 2021.
- Strosberg J, Gardner N, Kvols L. Survival and prognostic factor analysis of 146 metastatic neuroendocrine tumors of the mid-gut. Neuroendocrinology 2009;89(4):471–476.
- Banck MS, Kanwar R, Kulkarni AA, Boora GK, Metge F, Kipp BR, Zhang L, Thorland EC, Minn KT, Tentu R, Eckloff BW, Wieben ED, Wu Y, Cunningham JM, Nagorney DM, Gilbert JA, Ames MM, Beutler AS. The genomic landscape of small intestine neuroendocrine tumors. J. Clin. Invest. 2013;123(6):2502–2508.
- Sato Y, Hashimoto S, Mizuno K-I, Takeuchi M, Terai S. Management of gastric and duodenal neuroendocrine tumors. World J. Gastroenterol. 2016;22(30):6817–6828.
- Garbrecht N, Anlauf M, Schmitt A, Henopp T, Sipos B, Raffel A, Eisenberger CF, Knoefel WT, Pavel M, Fottner C, Musholt TJ, Rinke A, Arnold R, Berndt U, Plöckinger U, Wiedenmann B, Moch H, Heitz PU, Komminoth P, Perren A, Klöppel G. Somatostatin-producing neuroendocrine tumors of the duodenum and pancreas: incidence, types, biological behavior, association with inherited syndromes, and functional activity. Endocr. Relat. Cancer 2008;15(1):229–241.
- Tang LH, Basturk O, Sue JJ, Klimstra DS. A Practical Approach to the Classification of WHO Grade 3 (G3) Well-differentiated Neuroendocrine Tumor (WD-NET) and Poorly Differentiated Neuroendocrine Carcinoma (PD-NEC) of the Pancreas. Am. J. Surg. Pathol. 2016;40(9):1192–1202.
- Halfdanarson TR, Rabe KG, Rubin J, Petersen GM. Pancreatic neuroendocrine tumors (PNETs): incidence, prognosis and recent trend toward improved survival. Ann. Oncol. 2008;19(10):1727–1733.
- Cloyd JM, Poultsides GA. Non-functional neuroendocrine tumors of the pancreas: Advances in diagnosis and management. World J. Gastroenterol. 2015;21(32):9512–9525.
- Simon T, Riemer P, Detjen K, Di Domenico A, Bormann F, Menne A, Khouja S, Monjé N, Childs LH, Lenze D, Leser U, Jarosch A, Rossner F, Morkel M, Blüthgen N, Pavel M, Horst D, Capper D, Marinoni I, Perren A, Mamlouk S, Sers C. DNA methylation reveals distinct cells of origin for pancreatic neuroendocrine carcinomas (PanNECs) and pancreatic neuroendocrine tumors (PanNETs). Cold Spring Harbor Laboratory 2021:2020.06.12.146811.
- Pavel M, Öberg K, Falconi M, Krenning EP, Sundin A, Perren A, Berruti A, ESMO Guidelines Committee. Electronic address: clinicalguidelines@esmo.org. Gastroenteropancreatic neuroendocrine neoplasms: ESMO Clinical Practice Guidelines for diagnosis, treatment and follow-up. Ann. Oncol. 2020;31(7):844–860.
- Crona J, Skogseid B. GEP- NETS UPDATE: Genetics of neuroendocrine tumors. Eur. J. Endocrinol. 2016;174(6):R275–90.
- Lemmens I, Van de Ven WJ, Kas K, Zhang CX, Giraud S, Wautot V, Buisson N, De Witte K, Salandre J, Lenoir G, Pugeat M, Calender A, Parente F, Quincey D, Gaudray P, De Wit MJ, Lips CJ, Höppener JW, Khodaei S, Grant AL, Weber G, Kytölä S, Teh BT, Farnebo F, Thakker RV. Identification of the multiple endocrine neoplasia type 1 (MEN1) gene. The European Consortium on MEN1. Hum. Mol. Genet. 1997;6(7):1177–1183.
- Chandrasekharappa SC, Guru SC, Manickam P, Olufemi SE, Collins FS, Emmert-Buck MR, Debelenko LV, Zhuang Z, Lubensky IA, Liotta LA, Crabtree JS, Wang Y, Roe BA, Weisemann J, Boguski MS, Agarwal SK, Kester MB, Kim YS, Heppner C, Dong Q, Spiegel AM, Burns AL, Marx SJ. Positional cloning of the gene for multiple endocrine neoplasia-type 1. Science 1997;276(5311):404–407.
- Guru SC, Goldsmith PK, Burns AL, Marx SJ, Spiegel AM, Collins FS, Chandrasekharappa SC. Menin, the product of the MEN1 gene, is a nuclear protein. Proc. Natl. Acad. Sci. U. S. A. 1998;95(4):1630–1634.
- Balogh K, Rácz K, Patócs A, Hunyady L. Menin and its interacting proteins: elucidation of menin function. Trends Endocrinol. Metab. 2006;17(9):357–364.
- Alrezk R, Hannah-Shmouni F, Stratakis CA. MEN4 and CDKN1B mutations: the latest of the MEN syndromes. Endocr. Relat. Cancer 2017;24(10):T195–T208.
- Chasseloup F, Pankratz N, Lane J, Faucz FR, Keil MF, Chittiboina P, Kay DM, Tayeb TH, Stratakis CA, Mills JL, Hernández-Ramírez LC. Germline CDKN1B Loss-of-Function Variants Cause Pediatric Cushing’s Disease With or Without an MEN4 Phenotype. The Journal of Clinical Endocrinology & Metabolism 2020;105(6):1983–2005.
- Sei Y, Zhao X, Forbes J, Szymczak S, Li Q, Trivedi A, Voellinger M, Joy G, Feng J, Whatley M, Jones MS, Harper UL, Marx SJ, Venkatesan AM, Chandrasekharappa SC, Raffeld M, Quezado MM, Louie A, Chen CC, Lim RM, Agarwala R, Schäffer AA, Hughes MS, Bailey-Wilson JE, Wank SA. A Hereditary Form of Small Intestinal Carcinoid Associated With a Germline Mutation in Inositol Polyphosphate Multikinase. Gastroenterology 2015;149(1):67–78.
- Dumanski JP, Rasi C, Björklund P, Davies H, Ali AS, Grönberg M, Welin S, Sorbye H, Grønbæk H, Cunningham JL, Forsberg LA, Lind L, Ingelsson E, Stålberg P, Hellman P, Tiensuu Janson E. A MUTYH germline mutation is associated with small intestinal neuroendocrine tumors. Endocr. Relat. Cancer 2017;24(8):427–443.
- Calvete O, Reyes J, Zuñiga S, Paumard-Hernández B, Fernández V, Bujanda L, Rodriguez-Pinilla MS, Palacios J, Heine-Suñer D, Banka S, Newman WG, Cañamero M, Pritchard DM, Benítez J. Exome sequencing identifies ATP4A gene as responsible of an atypical familial type I gastric neuroendocrine tumour. Hum. Mol. Genet. 2015;24(10):2914–2922.
- Scarpa A, Chang DK, Nones K, Corbo V, Patch AM, Bailey P, Lawlor RT, Johns AL, Miller DK, Mafficini A, Rusev B, Scardoni M, Antonello D, Barbi S, Sikora KO, Cingarlini S, Vicentini C, McKay S, Quinn MCJ, Bruxner TJC, Christ AN, Harliwong I, Idrisoglu S, McLean S, Nourse C, Nourbakhsh E, Wilson PJ, Anderson MJ, Fink JL, Newell F, Waddell N, Holmes O, Kazakoff SH, Leonard C, Wood S, Xu Q, Nagaraj SH, Amato E, Dalai I, Bersani S, Cataldo I, Dei Tos AP, Capelli P, Dav\`i MV, Landoni L, Malpaga A, Miotto M, Whitehall VLJ, Leggett BA, Harris JL, Harris J, Jones MD, Humphris J, Chantrill LA, Chin V, Nagrial AM, Pajic M, Scarlett CJ, Pinho A, Rooman I, Toon C, Wu J, Pinese M, Cowley M, Barbour A, Mawson A, Humphrey ES, Colvin EK, Chou A, Lovell JA, Jamieson NB, Duthie F, Gingras MC, Fisher WE, Dagg RA, Lau LMS, Lee M, Pickett HA, Reddel RR, Samra JS, Kench JG, Merrett ND, Epari K, Nguyen NQ, Zeps N, Falconi M, Simbolo M, Butturini G, Van Buren G, Partelli S, Fassan M, Khanna KK, Gill AJ, Wheeler DA, Gibbs RA, Musgrove EA, Bassi C, Tortora G, Pederzoli P, Pearson JV, Waddell N, Biankin AV, Grimmond SM. Whole-genome landscape of pancreatic neuroendocrine tumours. Nature 2017;543(7643):65–71.
- Scarpa A. The landscape of molecular alterations in pancreatic and small intestinal neuroendocrine tumours. Ann. Endocrinol.2019;80(3):153–158.
- Francis JM, Kiezun A, Ramos AH, Serra S, Pedamallu CS, Qian ZR, Banck MS, Kanwar R, Kulkarni AA, Karpathakis A, Manzo V, Contractor T, Philips J, Nickerson E, Pho N, Hooshmand SM, Brais LK, Lawrence MS, Pugh T, McKenna A, Sivachenko A, Cibulskis K, Carter SL, Ojesina AI, Freeman S, Jones RT, Voet D, Saksena G, Auclair D, Onofrio R, Shefler E, Sougnez C, Grimsby J, Green L, Lennon N, Meyer T, Caplin M, Chung DC, Beutler AS, Ogino S, Thirlwell C, Shivdasani R, Asa SL, Harris CR, Getz G, Kulke M, Meyerson M. Somatic mutation of CDKN1B in small intestine neuroendocrine tumors. Nat. Genet. 2013;45(12):1483–1486.
- Jiao Y, Shi C, Edil BH, de Wilde RF, Klimstra DS, Maitra A, Schulick RD, Tang LH, Wolfgang CL, Choti MA, Velculescu VE, Diaz LA Jr, Vogelstein B, Kinzler KW, Hruban RH, Papadopoulos N. DAXX/ATRX, MEN1, and mTOR pathway genes are frequently altered in pancreatic neuroendocrine tumors. Science 2011;331(6021):1199–1203.
- Marinoni I, Kurrer AS, Vassella E, Dettmer M, Rudolph T, Banz V, Hunger F, Pasquinelli S, Speel E, Perren A. Loss of DAXX and ATRX Are Associated With Chromosome Instability and Reduced Survival of Patients With Pancreatic Neuroendocrine Tumors. Gastroenterology 2014;146(2):453–460.e5.
- Heaphy CM, De Wilde RF, Jiao Y, Klein AP, Edil BH, Shi C, Bettegowda C, Rodriguez FJ, Eberhart CG, Hebbar S, Offerhaus GJ, McLendon R, Rasheed BA, He Y, Yan H, Bigner DD, Oba-Shinjo SM, Marie SKN, Riggins GJ, Kinzler KW, Vogelstein B, Hruban RH, Maitra A, Papadopoulos N, Meeker AK. Altered telomeres in tumors with ATRX and DAXX mutations. Science 2011;333(6041):425.
- Han X, Chen W, Chen P, Zhou W, Rong Y, Lv Y, Li J-A, Ji Y, Chen W, Lou W, Xu X. Aberration of ARID1A Is Associated With the Tumorigenesis and Prognosis of Sporadic Nonfunctional Pancreatic Neuroendocrine Tumors. Pancreas 2020;49(4):514–523.
- Roy S, LaFramboise WA, Liu T-C, Cao D, Luvison A, Miller C, Lyons MA, O’Sullivan RJ, Zureikat AH, Hogg ME, Tsung A, Lee KK, Bahary N, Brand RE, Chennat JS, Fasanella KE, McGrath K, Nikiforova MN, Papachristou GI, Slivka A, Zeh HJ, Singhi AD. Loss of Chromatin-Remodeling Proteins and/or CDKN2A Associates With Metastasis of Pancreatic Neuroendocrine Tumors and Reduced Patient Survival Times. Gastroenterology 2018;154(8):2060–2063.e8.
- Simbolo M, Vicentini C, Mafficini A, Fassan M, Pedron S, Corbo V, Mastracci L, Rusev B, Pedrazzani C, Landoni L, Grillo F, Cingarlini S, Rindi G, Luchini C, Scarpa A, Lawlor RT. Mutational and copy number asset of primary sporadic neuroendocrine tumors of the small intestine. Virchows Arch. 2018;473(6):709–717.
- Kulke MH, Freed E, Chiang DY, Philips J, Zahrieh D, Glickman JN, Shivdasani RA. High-resolution analysis of genetic alterations in small bowel carcinoid tumors reveals areas of recurrent amplification and loss. Genes Chromosomes Cancer 2008;47(7):591–603.
- Andersson E, Swärd C, Stenman G, Ahlman H, Nilsson O. High-resolution genomic profiling reveals gain of chromosome 14 as a predictor of poor outcome in ileal carcinoids. Endocr. Relat. Cancer 2009;16(3):953–966.
- Samsom KG, van Veenendaal LM, Valk GD, Vriens MR, Tesselaar MET, van den Berg JG. Molecular prognostic factors in small-intestinal neuroendocrine tumours. Endocr Connect 2019;8(7):906–922.
- Yao J, Garg A, Chen D, Capdevila J, Engstrom P, Pommier R, Van Cutsem E, Singh S, Fazio N, He W, Riester M, Patel P, Voi M, Morrissey M, Pavel M, Kulke MH. Genomic profiling of NETs: a comprehensive analysis of the RADIANT trials. Endocr. Relat. Cancer 2019;26(4):391–403.
- Bottarelli L, Azzoni C, Pizzi S, D’Adda T, Silini EM, Bordi C, Rindi G. Adenomatous polyposis coli gene involvement in ileal enterochromaffin cell neuroendocrine neoplasms. Hum. Pathol. 2013;44(12):2736–2742.
- Karpathakis A, Dibra H, Pipinikas C, Feber A, Morris T, Francis J, Oukrif D, Mandair D, Pericleous M, Mohmaduvesh M, Serra S, Ogunbiyi O, Novelli M, Luong T, Asa SL, Kulke M, Toumpanakis C, Meyer T, Caplin M, Meyerson M, Beck S, Thirlwell C. Prognostic Impact of Novel Molecular Subtypes of Small Intestinal Neuroendocrine Tumor. Clin. Cancer Res. 2016;22(1):250–258.
- Karpathakis A, Dibra H, Thirlwell C. Neuroendocrine tumours: Cracking the epigenetic code. Endocrine-Related Cancer 2013;20(3). doi:10.1530/ERC-12-0338.
- Chan CS, Laddha SV, Lewis PW, Koletsky MS, Robzyk K, Da Silva E, Torres PJ, Untch BR, Li J, Bose P, Chan TA, Klimstra DS, Allis CD, Tang LH. ATRX, DAXX or MEN1 mutant pancreatic neuroendocrine tumors are a distinct alpha-cell signature subgroup. Nat. Commun. 2018;9(1):4158.
- Baldelli R, Barnabei A, Rizza L, Isidori AM, Rota F, Di Giacinto P, Paoloni A, Torino F, Corsello SM, Lenzi A, Appetecchia M. Somatostatin analogs therapy in gastroenteropancreatic neuroendocrine tumors: current aspects and new perspectives. Front. Endocrinol.2014;5:7.
- Rinke A, Müller H-H, Schade-Brittinger C, Klose K-J, Barth P, Wied M, Mayer C, Aminossadati B, Pape U-F, Bläker M, Harder J, Arnold C, Gress T, Arnold R, PROMID Study Group. Placebo-controlled, double-blind, prospective, randomized study on the effect of octreotide LAR in the control of tumor growth in patients with metastatic neuroendocrine midgut tumors: a report from the PROMID Study Group. J. Clin. Oncol. 2009;27(28):4656–4663.
- Susini C, Buscail L. Rationale for the use of somatostatin analogs as antitumor agents. Ann. Oncol. 2006;17(12):1733–1742.
- Palazzo M, Lombard-Bohas C, Cadiot G, Matysiak-Budnik T, Rebours V, Vullierme M-P, Couvelard A, Hentic O, Ruszniewski P. Ki67 proliferation index, hepatic tumor load, and pretreatment tumor growth predict the antitumoral efficacy of lanreotide in patients with malignant digestive neuroendocrine tumors. Eur. J. Gastroenterol. Hepatol. 2013;25(2):232.
- Caplin ME, Pavel M, Ćwikła JB, Phan AT, Raderer M, Sedláčková E, Cadiot G, Wolin EM, Capdevila J, Wall L, Rindi G, Langley A, Martinez S, Blumberg J, Ruszniewski P. Lanreotide in Metastatic Enteropancreatic Neuroendocrine Tumors. N. Engl. J. Med. 2014;371(3):224–233.
- Sansovini M, Severi S, Ambrosetti A, Monti M, Nanni O, Sarnelli A, Bodei L, Garaboldi L, Bartolomei M, Paganelli G. Treatment with the Radiolabelled Somatostatin Analog 177Lu-DOTATATE for Advanced Pancreatic Neuroendocrine Tumors. Neuroendocrinology 2013;97(4):347–354.
- Strosberg J, El-Haddad G, Wolin E, Hendifar A, Yao J, Chasen B, Mittra E, Kunz PL, Kulke MH, Jacene H, Bushnell D, O’Dorisio TM, Baum RP, Kulkarni HR, Caplin M, Lebtahi R, Hobday T, Delpassand E, Van Cutsem E, Benson A, Srirajaskanthan R, Pavel M, Mora J, Berlin J, Grande E, Reed N, Seregni E, Öberg K, Lopera Sierra M, Santoro P, Thevenet T, Erion JL, Ruszniewski P, Kwekkeboom D, Krenning E, NETTER-1 Trial Investigators. Phase 3 Trial of 177Lu-Dotatate for Midgut Neuroendocrine Tumors. N. Engl. J. Med. 2017;376(2):125–135.
- Pusceddu S, Verzoni E, Prinzi N, Mennitto A, Femia D, Grassi P, Concas L, Vernieri C, Lo Russo G, Procopio G. Everolimus treatment for neuroendocrine tumors: latest results and clinical potential. Ther. Adv. Med. Oncol. 2017;9(3):183–188.
- Yao JC, Shah MH, Ito T, Bohas CL, Wolin EM, Van Cutsem E, Hobday TJ, Okusaka T, Capdevila J, de Vries EGE, Tomassetti P, Pavel ME, Hoosen S, Haas T, Lincy J, Lebwohl D, Öberg K, RAD001 in Advanced Neuroendocrine Tumors, Third Trial (RADIANT-3) Study Group. Everolimus for advanced pancreatic neuroendocrine tumors. N. Engl. J. Med. 2011;364(6):514–523.
- Weatherstone K, Meyer T. Streptozocin-based chemotherapy is not history in neuroendocrine tumours. Target. Oncol. 2012;7(3):161–168.
- Szkudelski T. The mechanism of alloxan and streptozotocin action in B cells of the rat pancreas. Physiol. Res. 2001;50(6):537–546.
- Maggio I, Mollica V, Brighi N, Lamberti G, Manuzzi L, Ricci AD, Campana D. The functioning side of the pancreas: a review on insulinomas. J. Endocrinol. Invest. 2020;43(2):139–148.
- Raymond E, Dahan L, Raoul J-L, Bang Y-J, Borbath I, Lombard-Bohas C, Valle J, Metrakos P, Smith D, Vinik A, Chen J-S, Hörsch D, Hammel P, Wiedenmann B, Van Cutsem E, Patyna S, Lu DR, Blanckmeister C, Chao R, Ruszniewski P. Sunitinib malate for the treatment of pancreatic neuroendocrine tumors. N. Engl. J. Med. 2011;364(6):501–513.
- Wang YJ, Kaestner KH. Single-Cell RNA-Seq of the Pancreatic Islets--a Promise Not yet Fulfilled? Cell Metab. 2019;29(3):539–544.
- Muraro MJ, Dharmadhikari G, Grün D, Groen N, Dielen T, Jansen E, van Gurp L, Engelse MA, Carlotti F, de Koning EJP, van Oudenaarden A. A Single-Cell Transcriptome Atlas of the Human Pancreas. Cell Systems 2016;3(4):385–394.e3.
- Yang Z, Zhang L, Serra S, Law C, Wei A, Stockley TL, Ezzat S, Asa SL. Establishment and Characterization of a Human Neuroendocrine Tumor Xenograft. Endocr. Pathol. 2016;27(2):97–103.
- Drost J, van Jaarsveld RH, Ponsioen B, Zimberlin C, van Boxtel R, Buijs A, Sachs N, Overmeer RM, Offerhaus GJ, Begthel H, Korving J, van de Wetering M, Schwank G, Logtenberg M, Cuppen E, Snippert HJ, Medema JP, Kops GJPL, Clevers H. Sequential cancer mutations in cultured human intestinal stem cells. Nature 2015;521(7550):43–47.
- Drost J, van Boxtel R, Blokzijl F, Mizutani T, Sasaki N, Sasselli V, de Ligt J, Behjati S, Grolleman JE, van Wezel T, Nik-Zainal S, Kuiper RP, Cuppen E, Clevers H. Use of CRISPR-modified human stem cell organoids to study the origin of mutational signatures in cancer. Science 2017;358(6360):234–238.
- Matano M, Date S, Shimokawa M, Takano A, Fujii M, Ohta Y, Watanabe T, Kanai T, Sato T. Modeling colorectal cancer using CRISPR-Cas9-mediated engineering of human intestinal organoids. Nat. Med. 2015;21(3):256–262.
- Mutoh H, Fung BP, Naya FJ, Tsai MJ, Nishitani J, Leiter AB. The basic helix-loop-helix transcription factor BETA2/NeuroD is expressed in mammalian enteroendocrine cells and activates secretin gene expression. Proc. Natl. Acad. Sci. U. S. A. 1997;94(8):3560–3564.
- Gu C, Stein GH, Pan N, Goebbels S, Hörnberg H, Nave K-A, Herrera P, White P, Kaestner KH, Sussel L, Lee JE. Pancreatic beta cells require NeuroD to achieve and maintain functional maturity. Cell Metab. 2010;11(4):298–310.
- Kazanjian A, Wallis D, Au N, Nigam R, Venken KJT, Cagle PT, Dickey BF, Bellen HJ, Gilks CB, Grimes HL. Growth factor independence-1 is expressed in primary human neuroendocrine lung carcinomas and mediates the differentiation of murine pulmonary neuroendocrine cells. Cancer Res. 2004;64(19):6874–6882.
- Bjerknes M, Cheng H. Cell Lineage metastability in Gfi1-deficient mouse intestinal epithelium. Dev. Biol. 2010;345(1):49–63.
- Shroyer NF, Wallis D, Venken KJT, Bellen HJ, Zoghbi HY. Gfi1 functions downstream of Math1 to control intestinal secretory cell subtype allocation and differentiation. Genes Dev. 2005;19(20):2412–2417.
- Chen C, Fang R, Davis C, Maravelias C, Sibley E. Pdx1 inactivation restricted to the intestinal epithelium in mice alters duodenal gene expression in enterocytes and enteroendocrine cells. Am. J. Physiol. Gastrointest. Liver Physiol. 2009;297(6):G1126–37.
- Larsson LI, Madsen OD, Serup P, Jonsson J, Edlund H. Pancreatic-duodenal homeobox 1 -role in gastric endocrine patterning. Mech. Dev. 1996;60(2):175–184.
- Holland AM, Hale MA, Kagami H, Hammer RE, MacDonald RJ. Experimental control of pancreatic development and maintenance. Proc. Natl. Acad. Sci. U. S. A. 2002;99(19):12236–12241.
- Ahlgren U, Jonsson J, Jonsson L, Simu K, Edlund H. beta-cell-specific inactivation of the mouse Ipf1/Pdx1 gene results in loss of the beta-cell phenotype and maturity onset diabetes. Genes Dev. 1998;12(12):1763–1768.
- Terry NA, Walp ER, Lee RA, Kaestner KH, May CL. Impaired enteroendocrine development in intestinal-specific Islet1 mouse mutants causes impaired glucose homeostasis. Am. J. Physiol. Gastrointest. Liver Physiol. 2014;307(10):G979–91.
- Du A, Hunter CS, Murray J, Noble D, Cai C-L, Evans SM, Stein R, May CL. Islet-1 is required for the maturation, proliferation, and survival of the endocrine pancreas. Diabetes 2009;58(9):2059–2069.
- Liu J, Hunter CS, Du A, Ediger B, Walp E, Murray J, Stein R, May CL. Islet-1 regulates Arx transcription during pancreatic islet alpha-cell development. J. Biol. Chem. 2011;286(17):15352–15360.
- Ediger BN, Du A, Liu J, Hunter CS, Walp ER, Schug J, Kaestner KH, Stein R, Stoffers DA, May CL. Islet-1 Is essential for pancreatic β-cell function. Diabetes 2014;63(12):4206–4217.
- Ait-Lounis A, Baas D, Barras E, Benadiba C, Charollais A, Nlend Nlend R, Liègeois D, Meda P, Durand B, Reith W. Novel function of the ciliogenic transcription factor RFX3 in development of the endocrine pancreas. Diabetes 2007;56(4):950–959.
- Ait-Lounis A, Bonal C, Seguín-Estévez Q, Schmid CD, Bucher P, Herrera PL, Durand B, Meda P, Reith W. The transcription factor Rfx3 regulates beta-cell differentiation, function, and glucokinase expression. Diabetes 2010;59(7):1674–1685.
- Piccand J, Vagne C, Blot F, Meunier A, Beucher A, Strasser P, Lund ML, Ghimire S, Nivlet L, Lapp C, Petersen N, Engelstoft MS, Thibault-Carpentier C, Keime C, Correa SJ, Schreiber V, Molina N, Schwartz TW, De Arcangelis A, Gradwohl G. Rfx6 promotes the differentiation of peptide-secreting enteroendocrine cells while repressing genetic programs controlling serotonin production. Mol Metab 2019;29:24–39.
- Smith SB, Qu H-Q, Taleb N, Kishimoto NY, Scheel DW, Lu Y, Patch A-M, Grabs R, Wang J, Lynn FC, Miyatsuka T, Mitchell J, Seerke R, Désir J, Vanden Eijnden S, Abramowicz M, Kacet N, Weill J, Renard M-E, Gentile M, Hansen I, Dewar K, Hattersley AT, Wang R, Wilson ME, Johnson JD, Polychronakos C, German MS. Rfx6 directs islet formation and insulin production in mice and humans. Nature 2010;463(7282):775–780.
- Soyer J, Flasse L, Raffelsberger W, Beucher A, Orvain C, Peers B, Ravassard P, Vermot J, Voz ML, Mellitzer G, Gradwohl G. Rfx6 is an Ngn3-dependent winged helix transcription factor required for pancreatic islet cell development. Development 2010;137(2):203–212.
- Piccand J, Strasser P, Hodson DJ, Meunier A, Ye T, Keime C, Birling M-C, Rutter GA, Gradwohl G. Rfx6 maintains the functional identity of adult pancreatic β cells. Cell Rep. 2014;9(6):2219–2232.
- Sosa-Pineda B, Chowdhury K, Torres M, Oliver G, Gruss P. The Pax4 gene is essential for differentiation of insulin-producing beta cells in the mammalian pancreas. Nature 1997;386(6623):399–402.
- Prado CL, Pugh-Bernard AE, Elghazi L, Sosa-Pineda B, Sussel L. Ghrelin cells replace insulin-producing beta cells in two mouse models of pancreas development. Proc. Natl. Acad. Sci. U. S. A. 2004;101(9):2924–2929.
- St-Onge L, Sosa-Pineda B, Chowdhury K, Mansouri A, Gruss P. Pax6 is required for differentiation of glucagon-producing alpha-cells in mouse pancreas. Nature 1997;387(6631):406–409.
- Sander M, Neubüser A, Kalamaras J, Ee HC, Martin GR, German MS. Genetic analysis reveals that PAX6 is required for normal transcription of pancreatic hormone genes and islet development. Genes Dev. 1997;11(13):1662–1673.
- Oster A, Jensen J, Edlund H, Larsson LI. Homeobox gene product Nkx 6.1 immunoreactivity in nuclei of endocrine cells of rat and mouse stomach. J. Histochem. Cytochem. 1998;46(6):717–721.
- Henseleit KD, Nelson SB, Kuhlbrodt K, Hennings JC, Ericson J, Sander M. NKX6 transcription factor activity is required for alpha- and beta-cell development in the pancreas. Development 2005;132(13):3139–3149.
- Nelson SB, Schaffer AE, Sander M. The transcription factors Nkx6.1 and Nkx6.2 possess equivalent activities in promoting beta-cell fate specification in Pdx1+ pancreatic progenitor cells. Development 2007;134(13):2491–2500.
- Choi MY, Romer AI, Wang Y, Wu MP, Ito S, Leiter AB, Shivdasani RA. Requirement of the tissue-restricted homeodomain transcription factor Nkx6.3 in differentiation of gastrin-producing G cells in the stomach antrum. Mol. Cell. Biol. 2008;28(10):3208–3218.
- Hang Y, Yamamoto T, Benninger RKP, Brissova M, Guo M, Bush W, Piston DW, Powers AC, Magnuson M, Thurmond DC, Stein R. The MafA transcription factor becomes essential to islet β-cells soon after birth. Diabetes 2014;63(6):1994–2005.
- Du A, McCracken KW, Walp ER, Terry NA, Klein TJ, Han A, Wells JM, May CL. Arx is required for normal enteroendocrine cell development in mice and humans. Dev. Biol. 2012;365(1):175–188.
- Wilcox CL, Terry NA, Walp ER, Lee RA, May CL. Pancreatic α-Cell Specific Deletion of Mouse Arx Leads to α-Cell Identity Loss. PLoS One 2013;8(6):e66214.
- Ye DZ, Kaestner KH. Foxa1 and Foxa2 control the differentiation of goblet and enteroendocrine L- and D-cells in mice. Gastroenterology 2009;137(6):2052–2062.
- Sund NJ, Vatamaniuk MZ, Casey M, Ang SL, Magnuson MA, Stoffers DA, Matschinsky FM, Kaestner KH. Tissue-specific deletion of Foxa2 in pancreatic beta cells results in hyperinsulinemic hypoglycemia. Genes Dev. 2001;15(13):1706–1715.
- Elsayed AK, Younis I, Ali G, Hussain K, Abdelalim EM. Aberrant development of pancreatic beta cells derived from human iPSCs with FOXA2 deficiency. Cell Death Dis. 2021;12(1):103.
- Lee CS, Sund NJ, Behr R, Herrera PL, Kaestner KH. Foxa2 is required for the differentiation of pancreatic alpha-cells. Dev. Biol. 2005;278(2):484–495.
- Fernandez CJ, Agarwal M, Pottakkat B, Haroon NN, George AS, Pappachan JM. Gastroenteropancreatic neuroendocrine neoplasms: A clinical snapshot. World J. Gastrointest. Surg. 2021;13(3):231–255.