ABSTRACT
The brain integrates the response to a variety of signals of energy need and availability to match food intake with energy expenditure, thereby maintaining body weight stability. Early work with rodent models with disrupted energy balance (generally obesity) identified many hypothalamic genes and signaling pathways that impact energy homeostasis. More recent studies have identified hindbrain circuits that interact with peripheral metabolic signals and hypothalamic circuits to impact energy balance. Feeding, signals of energy utilization, and hormonal signals of energy stores (such as leptin) modulate gene expression and neurotransmission in specialized circuits within the hypothalamus and brainstem to control food intake. While many of these circuits also control energy expenditure, the effects on body weight that arise from alterations in energy expenditure are generally more modest than the effects of produced by changes in feeding. Although most of the mechanistic work that defined the systems that control energy balance utilized rodent models, these systems have human orthologs whose disruption produces phenotypes comparable to those observed in rodents, confirming their conserved function.
INTRODUCTION
Historically, obesity was thought to represent a disorder of voluntary behaviors, (albeit exacerbated by the ready availability of food and the reduced need for energy expenditure afforded by modern societies); many continue to hold this belief even today. In reality, we now understand that food intake and body weight represent biologically controlled homeostatic variables, much like blood pressure. This understanding flows from the discovery of spontaneously occurring single gene mutations that promote obesity independently of environmental alterations, along with the more recent description of human genetic variants that influence weight gain. Furthermore, research building upon these genetic observations has identified many of the biological systems that mediate the control of energy homeostasis, most of which reside in or converge on the central nervous system (CNS).
Changes in body weight reflect an alteration of energy balance, where energy intake (calories from eating or drinking) and energy expenditure (either as locomotor activity, basal metabolism, or thermogenesis) become unequal. For instance, food intake in excess of energy expenditure promotes the accretion of excess weight. Adipose tissue represents the major repository for ingested energy that exceeds immediate needs (1) and excess adipose tissue represents the hallmark of obesity.
The energy density of adipose tissue is nearly 10-fold greater than muscle (protein) or liver (glycogen) (2). The ability to store energy in adipose tissue protects against environmental vicissitudes that might result in starvation, fetal wastage, and the inability to provide sufficient breast milk to the young. Therefore, evolution has likely selected for genetic variants that favor energy storage and conservation. The existence of environments in which excess calories are readily available with minimum or no effort occurred very recently in human evolution, while the human genetic blueprint evolved under the opposite circumstance. Thus, the modern obesity epidemic may represent, at least in part, a physiologic mismatch between the evolutionary pressures that bias toward energy storage and the modern, nutrient- and calorie-rich environment.
The brain plays a central role in maintaining energy balance. CNS circuits continuously assess and integrate peripheral metabolic, endocrine and neuronal signals, and modulate both behaviors and peripheral metabolism to respond to acute and chronic needs (3). The brain modifies energy intake and expenditure to match energy demands on an ongoing homeostatic basis, establishing a metabolic “set-point”.
A BRIEF HISTORICAL PERSPECTIVE ON THE MECHANISMS THAT CONTROL ENERGY BALANCE
Role for the Hypothalamus
The description of Frölich syndrome (hyperphagic obesity and hypogonadism in patients with pituitary tumors) initially suggested that the pituitary gland might control energy balance (4). Others noted that pituitary tumors often impinge on the overlying hypothalamus, however, and suggested that the hypothalamus might represent the main modulator of feeding. Indeed, experiments by Hetherington and Ranson in 1940 demonstrated that lesions of the ventral medial portion of the hypothalamus increased feeding and promoted weight gain in rats, while lesions in the lateral hypothalamus led to decreased feeding and weight loss (5). In addition to demonstrating the importance of the hypothalamus to energy balance, these findings also led Eliot Stellar to suggest the concept of a “satiety center” situated in the ventral medial portion of the hypothalamus and a “hunger center” located in the lateral hypothalamus (6).
This two-center model also fits with the notion that two behavioral systems govern feeding: the incentive and reward value system that modulates the wanting and liking of food, and the satiety system that promotes meal termination (associated with the sensation of “fullness”). While these systems are physiologically and anatomically integrated, simplicity often dictates their description and study as distinct entities. We now understand that the meal-terminating systems in the brainstem as well as the brain reward circuits work in conjunction with the hypothalamus to mediate the overall control of food intake and energy homeostasis. Furthermore, recent studies have demonstrated greater anatomic heterogeneity in the hypothalamic systems that control energy balance than suggested by the simple two-center model, as well as revealing finer functional complexity- with distinct subsets of neurons in the hypothalamus controlling individual aspects of food intake and energy expenditure.
Genetic Models of Obesity Prove the Lipostatic Model of Energy Balance
Animals (including humans) maintain remarkably constant adipose triglyceride stores (7), suggesting that the brain and periphery must communicate to coordinate feeding and energy expenditure so as to maintain this balance. Around the same time that lesioning studies demonstrated the importance of the hypothalamus for the control of energy balance (5, 8), Kennedy proposed the lipostatic hypothesis of hunger: that an inhibitory factor produced by white adipose tissue in proportion to fat mass suppresses eating and body weight gain (9). He further suggested that lesions of the ventral medial hypothalamus increase food intake because of the removal of the site of action of the inhibitory signal from the fat.
A strain of mice displaying dramatic hyperphagia and obesity from the time of weaning arose spontaneously at the Jackson Laboratory in 1949-50; the autosomal recessive allele conveying this phenotype was designated obese (ob) (10). Sixteen years later, a phenotypically similar mouse was identified (11). The diabetic state of these latter animals (studied on the diabetes-prone coisogenic KsJ background) distinguished them from ob/ob mice (which had been studied on the relatively diabetes-resistant B6 background), leading to the diabetes (db) designation for the new mutation.
Seeking the molecular predicates of the lipostatic system posited by Kennedy (9) and Hervey (12), Douglas Coleman at Jackson Labs performed parabiosis studies coupling the circulation of ob/ob mice to either wild-type or db/db mice (13). While ob/ob mice became lean when joined to a wild type, they died of starvation when joined to a db/db mouse. These findings led Coleman to hypothesize the deficiency of a blood-borne body weight-regulating factor in ob/ob mice and the unresponsiveness of db/db mice to this factor. Specifically, he suggested that the ob locus produced the secreted factor while the db locus encoded its receptor (13,14). In 1994, the Friedman group at Rockefeller University positionally cloned the gene mutated in ob/ob mice and demonstrated that it encoded a secreted factor (which they termed “leptin”) produced primarily by adipocytes (15). Exogenous leptin rescued the phenotype of ob/ob (now, Lepob/ob) mice, and decreased feeding and body weight in wild-type animals. Soon thereafter, several groups cloned the leptin receptor (LepR) and demonstrated the disruption of the crucial “long” LepR isoform (LepRb) in db/db(Leprdb/db) mice (16–19).
The identification of leptin thus demonstrated the essential veracity of the lipostatic hypothesis. Interestingly, subsequent work has revealed a more complicated biology for leptin (whose absence sends a stronger signal than its excess (see below)), as well as suggesting the existence of additional factors that may contribute to the lipostatic control of food intake and energy balance.
THE HYPOTHALAMUS AND THE HYPOTHALAMIC MELANOCORTIN SYSTEM
The hypothalamus coordinates a host of homeostatic systems (e.g., sodium and water balance, reproduction, body temperature) in addition to energy balance. Given its need to coordinate these various functions, the hypothalamus must sense a broad array of nutrients, metabolites, hormones, and other factors (20). Of the many distinct nuclei (collections of neuronal cells) in the hypothalamus, the arcuate nucleus (ARC) plays a unique role in sensing peripheral signals. The ARC lies at the base of the hypothalamus adjacent to the median eminence (ME), a circumventricular organ that lies outside the blood brain barrier to permit direct sampling of the blood (20).
Importantly, the initial lesions of the ventral medial hypothalamus reported by Hetheringon and Ranson included the ARC, as well as the ventromedial hypothalamic nucleus (VMH), the dorsomedial hypothalamic nucleus (DMH), and the periventricular hypothalamic nucleus (PVH). Lesions of the VMH nucleus alone failed to recapitulate the hyperphagic obesity caused by the larger (original) ventral medial lesions (21), suggesting important potential roles in the control of energy balance for one or more of these other hypothalamic nuclei.
The Arcuate Nucleus
Its proximity to the ME, together with its projections to deeper hypothalamic areas involved in the control of feeding (e.g., the DMH, PVH and the lateral hypothalamic area (LHA)), suggest that the ARC serves to sense humoral signals and convey this information to downstream structures to modulate homeostatic systems (Figure 1). Indeed, the core of the CNS melanocortin system, which integrates peripheral signals of energy balance and modulates feeding and energy expenditure, lies in the ARC (22).
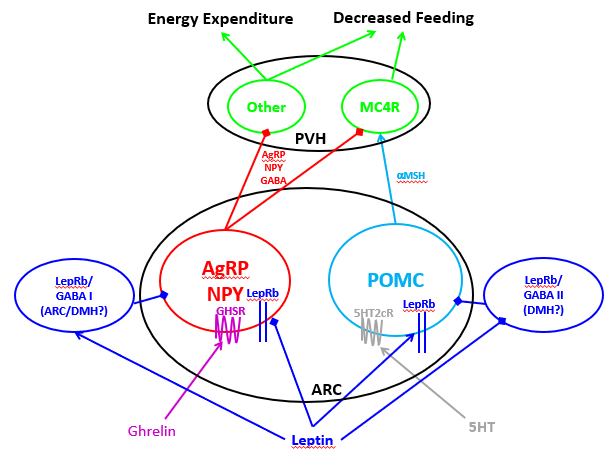
Figure 1. The hypothalamic melanocortin system. ARC POMC neurons produce aMSH and other POMC-derived peptides that act on downstream MC4R-expressing cells, such as PVH MC4R cells that play crucial roles in the suppression of food intake. ARC AgRP neurons (which also contain the inhibitory neurotransmitters NPY and GABA) release AgRP to antagonize MC4R signaling (increasing food intake) and also inhibit other PVH neurons to increase food intake and decrease energy expenditure. Signals of energy surfeit (including leptin) promote POMC neuron action; serotonin (5HT) also promotes POMC neuron action via 5HTR2c on these cells. In contrast, leptin inhibits AgRP cells, while orexigenic ghrelin also activates them. Not only does leptin act directly on these cells, but leptin action on unidentified LepRb/GABA neurons represents a major modulator of the melanocortin system.
Ay Reveals the Role for the CNS Melanocortin System in Energy Balance
In 1902, French geneticist L. Cuenot described the obese Yellow (Ay/a) mouse. Also termed ‘lethal yellow’ because homozygotes for Ay die before birth, Ay was bred by mouse fanciers in Europe beginning in the 1800s, and was notable for the dominant inheritance of a striking yellow coat, along with obesity proportional to the intensity of the yellowness of its coat (23). In 1960, another spontaneous mutation at the agouti locus arose in the Jackson Laboratory colony- viable yellow (Avy) (24). Expression of the wild-type agouti gene (a) normally occurs intermittently in the hair follicle, generating alternate yellow and black pigment bands of the resulting hair, producing the agouti coat color (25). The original Ay mutation represents a deletion within the gene encoding the RNA-binding protein Raly (Raly), which fuses the constitutively active Raly promoter to the agouti gene, resulting in constitutive ectopic overexpression of agouti in all somatic (including brain) cells (26). Avy also results from ectopic overexpression of agouti- due to the insertion of a retrovirus-like repetitive intracisternal A particle (IAP) into a noncoding exon of agouti (27).
The agouti locus encodes agouti signaling protein (ASP), a peptide with high affinity for melanocortin receptors. The yellow coat color of the Ay/a mouse results from continuous overexpression of ASP in the skin, which blocks alpha-melanocyte-stimulating hormone (α-MSH) signaling at melanocortin-1 receptors (MC1R) in the hair follicle (25,28). Since α-MSH activates melanocytes to initiate the synthesis of eumelanin (black pigment) instead of phaeomelanin (yellow pigment), antagonism of α-MSH/MC1R signaling by ASP elicits a yellow coat color.
The brain also contains a melanocortin system, and this CNS melanocortin system controls energy balance (22). ICV administration of α-MSH or other melanocortin agonists decreases food intake and body weight (29). Overexpression of ASP in the Ay/a brain antagonizes the anorectic action of α-MSH signaling and blunts the activity of brain melanocortin receptors, thus causing hyperphagia.
Melanocortin Peptides and Receptors
The post-translational modification and cleavage of the proopiomelanocortin (POMC) precursor peptide produces several melanocortin peptides, including adrenocorticotrophic hormone (ACTH), α-MSH (more prominent in rodents), ß-MSH (more prominent in humans) and γ-MSH; POMC processing also produces the opioid peptide, ß-endorphin (22). Within the CNS, the major population of POMC-producing cells resides in the ARC (a smaller population of brainstem POMC neurons may produce low levels of POMC and plays unclear roles in brain melanocortin signaling) (22). CNS melanocortin peptides act via the melanocortin-3 and -4 receptors (MC3R and MC4R) on target neurons. The ARC also contains neurons that produce agouti-related protein (AgRP, an antagonist/inverse agonist for MC3R and MC4R), along with the inhibitory neurotransmitters neuropeptide Y (NPY) and gamma amino butyric acid (GABA) (30),(31). Thus, the core of the CNS melanocortin system comprises anorexigenic (appetite–suppressing) ARC POMC neurons, opposing orexigenic (hunger-inducing) ARC AgRP neurons, and MC3R and MC4R-containing target neurons throughout the CNS (22) (Figure 1).
ARC POMC Neurons
Signals of positive energy balance, such as leptin, tend to activate POMC neurons and increase their Pomcexpression (32). Artificially activating ARC POMC neurons decreases food intake (33,34). While ARC POMC neurons also contain the neuropeptide CART (and a few POMC neurons contain various amino acidergic transmitters) (35,36), most data suggest that melanocortin peptide action mediates the majority of the POMC neurons’ ability to suppress food intake and increase energy expenditure (37). The ablation of ARC Pomc expression promotes hyperphagic obesity similar to that of Ay mice (34),(38). The first evidence for a human melanocortin obesity syndrome resulted from the astute recognition of a rare agouti-mouse–like syndrome in two families, resulting from null mutations in the POMC gene (39–41). These patients have ACTH insufficiency, red hair, and obesity, resulting from the lack of ACTH peptide in the serum and a lack of melanocortin peptides in skin and brain, respectively. This obesity syndrome demonstrated that the CNS melanocortin circuitry subserves energy homeostasis in humans as it does in the mouse.
The predictable, monogenetic heritability of the hyperphagic and obese phenotype caused by Ay, ob, and dbdemonstrates the genetic underpinnings of feeding control and overall energy balance. The subsequent finding that the orthologs of rodent obesity genes control body weight in humans confirms that biologic/genetic factors control feeding and the predisposition to obesity in humans, as well as in rodents (42).
ARC AgRP Neurons
Fasting and signals indicating negative energy balance activate ARC AgRP neurons, while signals of positive energy balance (e.g., leptin) inhibit these cells. ARC AgRP neuron activation promotes feeding and decreases energy expenditure, while neuronal ablation results in lethal anorexia, consistent with the strong orexigenic nature of these cells (43,44). AgRP acts as an inverse agonist at MC3/4R, decreasing receptor activity and thus promoting positive energy balance by increasing food intake and decreasing energy expenditure (25). While the ablation of Agrp and/or Npy in ARC AgRP neurons minimally affects energy balance in wild-type animals, it attenuates the obesity of leptin-deficient animals (45). In contrast, blockade of GABA release from these neurons, via the cre recombinase-mediated deletion of the vesicular GABA transporter (vGat), results in leanness and interferes with the response to food restriction, suggesting that these neurons (and especially GABA release therefrom) are crucial for promoting food intake, especially in response to signals of negative energy balance (46). Importantly, the ARC contains additional populations of (non-AgRP-containing) GABA neurons that may mediate orexigenic signals in a manner similar to AgRP cells (47).
Downstream Targets of the ARC Melanocortin System
Melanocortin-mediated stimulation of MC3/4R decreases food intake and increases energy expenditure to promote negative energy balance in animals and humans (48–50). Mice null for Mc4r display substantial hyperphagia and increased adiposity/body weight, and also display increased linear growth, as is characteristic of Ay/a mice (51). Mc3r-null mice display a more modest energy balance phenotype than Mc4r-null mice, with only modestly increased adipose mass, decreased lean mass, reduced fast-induced refeeding (52,53), elevated basal and fasting-induced corticosterone (53), and defects in circadian rhythms and meal entrainment (54). Thus, MC4R represents the major melanocortin receptor that mediates the control of food intake and body weight. Regions that contain large populations of MC3R- and MC4R-expressing neurons include the PVH, LHA, DMH, VMH, and ARC (the VMH and ARC contain MC3R only) (55).
While a syndrome resulting from MC3R mutations in humans has not yet been definitively identified, MC4R clearly plays an important role in the control of body weight in humans, as well. Heterozygous frameshift mutations in the human MC4R locus associate with physical findings virtually identical to those reported for the mouse (51), with increased adipose mass, increased linear growth and lean mass, hyperinsulinemia greater than that seen in matched obese control subjects, and severe hyperphagia. MC4R haploinsufficient adults also exhibit reduced sympathetic tone and mild hypotension (56). MC4R haploinsufficiency in humans represents the most common monogenic cause of severe obesity, accounting for up to 5% of cases (57–59).
Site-specific deletion studies have demonstrated a crucial role for MC4R in the PVH for the control of food intake and energy balance (60,61). While AgRP neurons project to and inhibit ARC POMC neurons via direct GABA action (62), this projection appears to play little role in the promotion of feeding by AgRP neurons (63). Rather, AgRP neurons most strongly increase feeding via their projections to the PVH (LHA projections also participate)(64). Thus, the PVH plays crucial roles in the control of feeding by POMC and AgRP neurons.
Interestingly, while AgRP neuron activation promotes feeding most strongly via the PVH, AgRP neuron inhibition decreases food intake at a distinct site: detailed studies of animals ablated for AgRP neurons demonstrate that the withdrawal of GABAergic inhibition from cells in the brainstem parabrachial nucleus (PBN) mediate this affect (65)(See below for additional details).
Paraventricular Nucleus of the Hypothalamus (PVH)
The PVH represents a major output nucleus for the hypothalamus, from which integrated information is transmitted to effector systems, such as the pituitary gland, the autonomic system, and behavioral control circuits (66,67). The identification of small deletions or translocations at the human Single-minded-1 (SIM1) locus on chromosome 6 in three young obese patients suggested a crucial role for the PVH in energy balance in humans (68). SIM1 encodes a transcription factor that is expressed throughout the PVH and is required for the development of the PVH (68). While homozygous deletion of Sim1 is embryonic lethal in mice, animals heterozygous for Sim1 are normal until 4 weeks of age, when they develop hyperphagic obesity (69). These mice display reduced numbers of neuronal nuclei in the PVH with a proportional decrease in overall size of the PVH. Presumably, the decreased number of PVH neurons in Sim1haploinsufficiency diminishes anorexic “tone” from the PVH, leading to hyperphagia and obesity in mice as well as in rare human patients with SIM1 mutations.
As with other hypothalamic nuclei, the PVH contains a constellation of diverse neuronal subtypes. Identifying the PVH subpopulations that mediate effects on food intake and energy expenditure represent a crucial research direction. Unsurprisingly, PVH MC4R neurons potently suppress food intake (60,61,70). Interestingly, however, PVH-projecting ARC AgRP neurons regulate cells that lack MC4R (in addition to regulating MC4R neurons), suggesting the existence of additional PVH populations that play roles in the control of energy balance (71). Nos1-expressing PVH cells represent one important subset of appetite-regulating non-MC4R PVH cells (72). Other important non-MC4R PVH neurons include prodynorphin (Pdyn)-expressing cells (71).
Prominent populations of PVH neurons include those that contain hormones/neuropeptides, including oxytocin (OXT),corticotropin releasing hormone (CRH), and thyrotropin releasing hormone (TRH), arginine vasopressin (AVP), and oxytocin (OXT) (61,64,70,73).These peptides also control other endocrine and CNS functions: TRH and CRH stimulate the thyroid and adrenal axes, respectively; AVP contributes to fluid balance; and OXT regulates uterine function and social interactions (74–78). While these peptidergic PVH neurons do not contain MC4R, the injection of OXT into the hindbrain promotes satiation (64). Genetic data from mice argue against an important role of OXT or OXT neurons in energy balance, however. Not only do Oxt-null animals display no alteration in feeding or energy balance, but neither the activation nor the ablation of PVH OXT neurons in adult animals alters food intake (72,79). Furthermore, all of these peptide-containing PVH populations are only weakly anorexigenic in mice, and OXT, AVP, and CRH neurons do not mediate melanocortin responses (61). Thus, peptidergic PVH neurons play little role in the control of feeding, at least in mice, while distinct Mc4r-, Nos1-, and Pdyn-containing PVH neurons (along with potentially other PVH neuron types that will be important to identify) play crucial roles in the control of feeding and energy balance. Interestingly, a recent GWAS analysis identified a polymorphism near the human anaplastic lymphoma kinase (ALK) locus that correlates with thinness. Decreased expression of this gene reduces adiposity in a variety of animal models and Alk expression in the PVH appears to mediate its effects on body weight (80). Identifying the cell type(s) that mediate the effects of Alk on body weight will be very informative.
Dorsomedial Nucleus of the Hypothalamus (DMH)
The DMH has long been implicated in energy balance regulation, as well as in the modulation of body temperature, arousal and circadian rhythms of locomotor activity (81). This nucleus receives direct input from the ARC and also contains LepRb-expressing neurons (82,84). While the exact molecular phenotype(s) of energy balance-regulating DMH cells remain poorly defined, recent studies have suggested that the LepRb-containing cells in this region play crucial roles for maintaining energy balance (85). Indeed, the viral-mediated disruption of DMH LepRb in adult mice augments food intake and promotes obesity (86). Furthermore, subpopulations of GABAergic DMH neurons play important roles in the leptin-mediated control of ARC POMC and AgRP cells (and thus, food intake) (85,87,88). TrkB-containing DMH neurons also contribute to the control of homeostatic feeding behavior (89). Thus, while details continue to emerge, the DMH plays crucial roles in leptin action, the control of the hypothalamic melanocortin system, food intake, and overall energy balance.
Ventromedial Nucleus of the Hypothalamus (VMH)
The VMH contains neurons that express LepRb, MC3R and other receptors involved in body weight regulation. Neurons in the dorsomedial portion of the VMH (dmVMH) express the transcription factor, steroidogenic factor 1 (Sf1; Nr5a1) (90). Although Sf1-deficient mice were first described in 1994, their early death due to adrenal insufficiency initially prevented the study of these mice in adulthood. Later, adrenal transplantation enabled the long-term survival of these mice, permitting the detection of late-onset obesity in Sf1-deficient mice (91), consistent with a role for the VMH in the control of energy balance. The obesity of Sf1-null mice results largely from decreased energy expenditure, however (91). Furthermore, Sf1-cre-mediated ablation of LepRb doesn’t alter food intake, but rather decreases energy expenditure (thereby accentuating obesity in high-fat diet-fed animals) (92). Many Sf1-containing VMH neurons contain the neuropeptide PACAP (the product of the Adcyap gene), which contributes to the control of energy expenditure (93). Thus, Sf1-mediated manipulation of the dorsomedial VMH has revealed a crucial role for this region in overall energy balance, albeit by the modulation of energy expenditure, rather than food intake. Indeed, the dmVMH is generally thought to serve as an autonomic control center that modulates a variety of parameters driven by the sympathetic nervous system (SNS). In addition to controlling energy expenditure, the dmVMH also plays important roles in nutrient mobilization (as during the response to hypoglycemia) (94–97).
Lateral Hypothalamic Area (LHA)
While a network of systems that suppress food intake (albeit in a manner antagonized by AgRP neurons) reside in the ARC, DMH, and PVH, the LHA is often thought of as a region that promotes feeding. Well-known LHA neuronal subtypes include two distinct sets of excitatory neurons that receive input from leptin and melanocortins and contribute to the control of feeding and energy balance. One population contains the neuropeptide melanin concentrating hormone (MCH; not related to POMC or any of its derivative peptides) (98). First studied in mammals because of the increased expression of Mch mRNA in Lepob/ob and fasted mice, administration of MCH increases food intake and body weight gain and decreases energy expenditure(98). Furthermore, animals null for Mch (or its receptor) are lean (99). The MCH receptor localizes to the primary cilium, and some of the effects of ciliopathies on adiposity may be conveyed by effects on this receptor (see discussion of ciliopathies below).
A distinct set of LHA neurons contain the neuropeptide, hypocretin (HCRT; also known as orexin) (100,101). Based upon early acute pharmacologic studies, HCRT was originally conceived of as an orexigen, since HCRT stimulates food intake when injected centrally during the light cycle. Consistently, fasting increases Hcrt mRNA expression and activates HCRT neurons (101). Subsequent work has revealed that animals null for HCRT or its receptors become mildly obese without observable alterations in food intake, however (102). Furthermore, mice (and dogs and humans) null for Hcrt or lacking HCRT neurons exhibit narcolepsy and increased body weight and adiposity (103). Thus, rather than having a primary role in the control of feeding, HCRT neurons promote alertness and activity, and most of the effect of Hcrt mutation on energy balance results from decreased physical activity and energy expenditure, while HCRT administration promotes activity (and food intake) during the resting phase of the diurnal cycle.
The LHA also contains LepRb neurons that control HCRT neurons; these contain neurotensin and lie intermingled with the HCRT cells (104-107). Ablation of LepRb from these LHA cells prevents the normal regulation of HCRT neurons and results in decreased locomotor activity and energy expenditure. Both LHA LepRb neurons and HCRT cells project to the ventral tegmental area (VTA), which contains a large number of dopaminergic neurons that represent the core of the mesolimbic reward system (see below for further discussion of reward pathways). Thus, while lesioning studies suggest that the integrity of the LHA is required for motivation and normal feeding behavior, most data suggest that it plays little role in the normal modulation of food intake.
PERIPHERAL SIGNALS THAT MODULATE ENERGY BALANCE VIA THE HYPOTHALAMUS
Homeostatic regulation of energy balance requires the brain to maintain appropriate energy levels by monitoring peripheral signals of energy status and metabolism to modulate food intake and a variety of autonomic and neuroendocrine determinants of energy utilization. This requires the ability to sense circulating signals of metabolic status.
Leptin
The discovery of leptin revealed the existence of an endocrine system that senses and modulates adipose stores. Disruption of leptin signaling results in hyperphagia and obesity, and leptin administration to leptin-deficient Lepob/obmice (but not LepRb-null Leprdb/db animals), reduces food intake and adiposity, sparing lean tissue (108–110). While the role for leptin in the control of appetite and adiposity initially dominated the thinking about its biology, it has become clear that the effects of elevated leptin are not as dramatic as those of low leptin. Indeed, diet-induced obese rodents and humans remain obese despite exhibiting high circulating concentrations of leptin, commensurate with their high levels of leptin-producing adipose tissue (111,112). In contrast to the Lepob/ob mice, where leptin administration results in remarkable reversal of the obesity phenotype, increasing leptin to supraphysiologic levels in normal animals only modestly and briefly blunts food intake and body weight. Likewise, supraphysiological doses of leptin promote only modest effects on body weight in obese and non-obese humans(113). Thus, the absence of leptin conveys a more powerful signal than does its excess.
Lepob/ob mice (and their leptin-deficient human counterparts) display additional phenotypes, including impaired growth and gonadal axis function, diminished immune function, infertility, and decreased activity and energy expenditure - all of which are reversed by leptin treatment (114,115). The lack of leptin also promotes increased hepatic glucose production, and leptin treatment suppresses hyperglycemia in several models of insulinopenic diabetes (116,117). Lipodystrophic people and transgenic animals that similarly lack adipose tissue exhibit leanness and low leptin levels, as well as hyperphagia, insulin resistance, diabetes and other endocrine and metabolic abnormalities that are not corrected by caloric restriction (109,110,118). Leptin replacement therapy to correct low leptin concentrations represents an important treatment for lipodystrophy syndromes in humans, decreasing their hunger and improving their endocrine and metabolic abnormalities (119).
This constellation of phenotypes resulting from low leptin mirrors the physiologic response to starvation and leptin treatment attenuates many of these consequences of very low adiposity (115). Thus, normal leptin concentrations signal the repletion of energy (fat) stores to mitigate hunger and enable energy expenditure, while low leptin indicates the dearth of adipose reserves and promotes food-seeking and the conservation of remaining fat by reducing energy expenditure.
THE NEUROBIOLOGY OF LEPTIN
The similar phenotypes of Lepob/ob and Leprdb/db mice (along with the inability of leptin to alter physiology in Leprdb/dbmice) indicates that leptin action on LepRb-expressing cells must mediate its effects. Consistent with its behavioral effects (e.g., on feeding) and its effects on the neuroendocrine and autonomic systems, most LepRb-expressing cells lie in the brain (83,84). Similarly, ablation of LepRb in the CNS promotes hyperphagia, neuroendocrine failure, and obesity (120). Some cells outside of the CNS might express LepRb, but the physiologic role for leptin action on these non-CNS cells remains unclear.
Within the brain, the majority of LepRb-expressing neurons reside within the hypothalamus and brainstem, consistent with the known roles for these structures in the control of feeding, endocrine and autonomic function (83,84,121). Pan-hypothalamic ablation of LepRb promotes a phenotype very similar in quality and magnitude to that of Leprdb/dbanimals (122). Furthermore, ablation of LepRb from broadly-distributed hypothalamic vGat- or Nos1-expressing neurons promotes dramatic hyperphagia and obesity (123,124). Smaller, more circumscribed sets of hypothalamic LepRb neurons have also been implicated in body weight control as well. Within the ARC, early developmental removal of LepRb specifically in POMC and AgRP neurons modestly increases feeding and adiposity (125,126). Interestingly, removal of LepRb from AgRP neurons in adult animals results in robust hyperphagia, obesity and diabetes, suggesting that developmental processes can largely compensate for the early lack of direct leptin action on AgRP neurons (127). Ablation of LepRb in the Sf1-expressing VMH blunts the increase in energy expenditure that accompanies increased adiposity, and deletion of LepRb in the LHA diminishes motor activity and promotes obesity (92,106,128). LepRb neurons in the ventral premammillary nucleus (PMv) play roles in reproduction (129). Importantly, functions for many additional groups of LepRb cells in the hypothalamus (especially in the DMH) have yet to be determined. Currently, LepRb neurons in the ARC and DMH are thought to play the most important roles in the control of feeding and energy balance by leptin.
THE MOLECULAR BIOLOGY OF LEPTIN
Alternative splicing of the Lepr transcript produces multiple isoforms of the receptor: LepRa, -b, -c, -d, etc (Figure 2). The Leprdb mutation mouse results from a splicing defect that causes the LepRa-specific exon to be inserted into the mRNA that encodes LepRb, preventing translation of the LepRb-specific coding sequences and producing LepRa in place of LepRb (16–18). Because the Leprdb/db mouse synthesizes all leptin receptor isoforms except LepRb, LepRb must be crucial for the control of energy homeostasis (130). Indeed, restoration of LepRb on a background null for all other LepR isoforms restores energy balance (19).
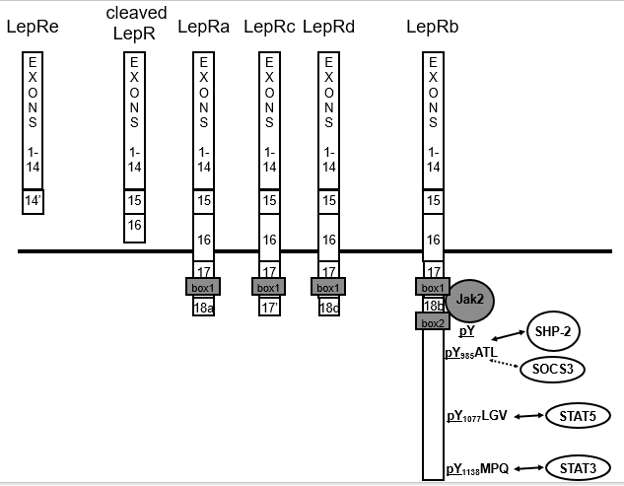
Figure 2. LepR isoforms and signaling. LepRa (Ra) represents the mostly highly expressed short form of LepR; LepRb (Rb) is the long form. Exon 17 contains half of a Jak docking site (BOX1) common to Ra, Rb and Rc, while exon 18b contains additional motifs required for full Jak2 binding (BOX2) and STAT3 signaling (31,33). Circulating leptin binding protein consists of extracellular domain that has been cleaved from the cell surface, along with the LepRe splice variant that lacks a transmembrane domain. Humans do not generate the splice variant, so that all LepRe is produced by cell surface cleavage, presumably by membrane associated metalloproteases (33). LepRa, -c, -d and the other so-called “short” isoforms contain the same first 17 exons as LepRb, but diverge within the intracellular domain. LepRb is the only isoform that mediates classical Jak-STAT signaling, as this isoform alone contains the motifs required to interact with Jak2 and to bind STAT proteins for downstream signaling (Figure 1) (34). While the function of LepRb is clear, the functions of the short isoforms are not, although they have been speculated to function in leptin transport into the brain and/or a source of cleaved, circulating extracellular LepR (which, along with LepRe comprises the major circulating leptin-binding protein) (35).
LepRb, like other type 1 cytokine receptors, activates a JAK family tyrosine kinase (JAK2) to initiate signaling (130). Subsequently, tyrosine phosphorylated residues on LepRb recruit STAT proteins, which are then phosphorylated by JAK2 to promote their trafficking to the nucleus. In the nucleus, STATs bind DNA and modulate gene expression. STAT3 mediates the majority of leptin action, since disruption of the binding site for STAT3 on LepRb causes a severe obesity phenotype in mice that is similar to the obesity syndrome of Leprdb/db mice (131). Similarly, disruption of Stat3in the forebrain or in LepRb-expressing neurons results in obesity in mice (132,133). While the brain-wide disruption of the genes encoding both isoforms of STAT5 (STAT5a and STAT5b) causes mild late-onset obesity, the disruption of Stat5a/b specifically in LepRb neurons produces no detectable phenotype, suggesting that STAT5 signaling is not required for leptin action in vivo (134–136). STAT5 represents a major mediator of GM-CSF signaling, however, and mice null for GM-CSFR in the brain are obese, suggesting that the role for STAT5 in energy balance may be linked to the action of GM-CSF or other cytokines different than leptin (135).
Insulin
Like leptin, insulin circulates in proportion to fat mass, and alters neuropeptide expression in the hypothalamus via receptors located in the ARC, PVH, and DMH (137). ICV insulin has been reported to decrease food intake in rats and mice. Furthermore, mice deleted for insulin receptor (Insr) throughout the CNS display a modest late-onset obesity (more prominent in females), and are more susceptible to diet-induced obesity than wild-type mice (138). In addition, insulin acts centrally to decrease hepatic glucose output, in part via the inhibition of AgRP neurons (139,140).
The insulin receptor (INSR), a tyrosine kinase, recruits and tyrosine phosphorylates insulin receptor substrates (IRS proteins; IRS-1, -2, -3, -4) which engage downstream signals, including the phosphatidylinositol 3-kinase (PI3-kinase) pathway. Deletion of Irs1 interferes primarily with peripheral insulin action and the growth axis, Irs3 is rodent-specific and adipocyte-restricted, and the deletion of Irs4 minimally alters energy balance (141). In contrast, deletion of Irs2causes insulin-deficient diabetes (due to islet failure) and obesity. Restoration of Irs2 in the islets of Irs2-null mice or brain-specific ablation of Irs2 results in normoglycemic obesity, consistent with a role for brain IRS2 signaling in energy balance (142). While leptin modulates the IRS-protein/PI3-kinase pathway and the deletion of Irs2 from LepRb-expressing neurons promotes obesity (albeit a milder form of obesity than observed in animals deleted for Irs2throughout the brain), deletion of Irs2 does not interfere with leptin action, suggesting that IRS2 may primarily play a role in brain insulin action (143).
A variety of subunits and downstream effectors of the PI3-kinase signaling pathway have also been deleted in several neuronal populations in mice (144). These produce phenotypes generally consistent with the notion that PI3-kinase is important for the proper function of the POMC and AgRP neurons that modulate energy balance- at least in part by controlling the firing of these important neurons.
Modulators of Insulin and Leptin Signaling
Many of the molecular signaling pathways that inhibit insulin and leptin action overlap. Protein tyrosine phosphatase-1B (PTP1B, a.k.a., PTPN1) dephosphorylates cognate tyrosine kinases (including those associated with INSR and LepRb) to terminate signaling (145,146). In addition to exhibiting increased insulin sensitivity, mice lacking Ptpn1 are lean compared to controls and exhibit resistance to weight gain on a high-fat diet, suggesting increased leptin action in these animals. Indeed, animals null for Ptpn1 throughout the brain (or specifically in LepRb or POMC neurons) demonstrate increased leanness and enhanced leptin action (147,148). In addition to PTP1B, the tyrosine phosphatase, TCPTP, which directly dephosphorylates STAT3, contributes to the attenuation of LepRb signaling. Furthermore, obesity and elevated leptin increase the expression of Ptpn2 (which encodes TCPTP), and the deletion of neuronal Ptpn2 decreases body weight, increases leptin sensitivity, and blunts weight gain in DIO animals (149). Moreover, the combined deletion of Ptpn1 and Ptpn2 in the brain augments leanness and further attenuates weight gain in DIO mice (149).
Suppressors of Cytokine Signaling (SOCS proteins, e.g., SOCS1 and SOCS3) bind to activated cytokine receptor/Jak2 kinase complexes (including the LepRb/Jak2 complex) to mediate their inhibition and degradation (150). SOCS proteins may also inhibit INSR and other related tyrosine kinases. Leptin signaling via STAT3 promotes Socs3expression in hypothalamic LepRb neurons; SOCS3 protein binds to phosphorylated Tyr985 of LepRb to attenuate LepRb signaling (151). The leanness of mice containing a substitution mutation of LepRb Tyr985 and the similar phenotype of mice lacking Socs3 in the brain or in LepRb neurons highlight the importance of these mechanisms of feedback inhibition for the control of energy balance (152,153). While LepRb Tyr985 also mediates the recruitment of the tyrosine phosphatase SHP2 (aka, PTPN11), data from cultured cells suggest that SHP2 mediates ERK pathway signaling by LepRb, and disruption of Ptpn11 in the brain, in LepRb neurons, or in POMC neurons, promotes obesity (130) (Figure 3).
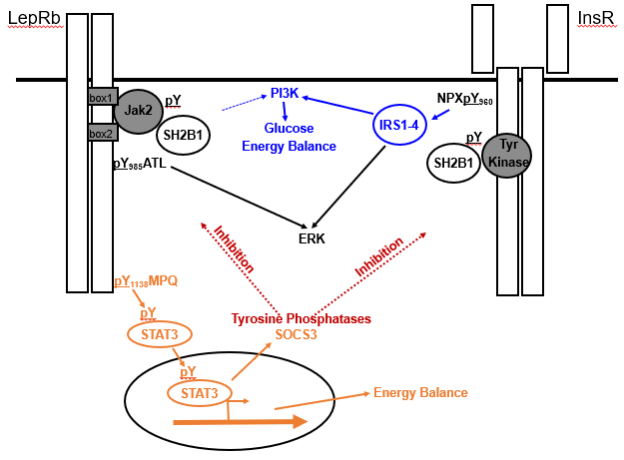
Figure 3. Signaling by and inhibition of LepRb and InsR. LepRb, which exists as a preformed homodimer in complex with the Jak2 tyrosine kinase, recruits and phosphorylates (pY) STAT3 via phosphorylated pY1138 to control many aspects of energy balance. InsR, which also exists as a preformed dimer, but has intrinsic tyrosine kinase activity, autophosphorylates the juxtamembrane Tyr960 to recruit the insulin receptor substrate (IRS) proteins IRS1-IRS4. IRS-proteins strongly activate the phosphatidylinositol 3-kinase (PI3K), which play roles in the brain control of energy balance and glucose homeostasis. Leptin also activates PI3K, albeit much more weakly than InsR, and by undefined mechansims. Both LepRb and InsR activate the ERK pathway. The adapter protein, SH2B1 also enhances signaling by both receptors. In addition to decreasing food intake and increasing energy expenditure, LepRb-mediated STAT3 signaling promotes the expression of SOCS3, which acts as a feedback inhibitor of LepRb and InsR signaling. A variety of tyrosine phosphatases also inhibit the activity of both receptors.
SH2B1 binds to activated Jak2, as well as to INSR, TrkB, and a few other receptor tyrosine kinase complexes to increase their activity and mediate aspects of downstream signaling (154). Sh2b1-null mice display a complex phenotype that includes obesity; brain-specific absence of Sh2b1 also promotes obesity in mice (155,156). Thus, SH2B1 signaling in the brain is required for energy balance, perhaps due to its requirement for correct signaling by multiple receptors involved in energy homeostasis. Furthermore, the phenotype of several human patients with morbid obesity, developmental delay, and behavioral disorders are associated with chromosomal deletions (16p11.2) or coding variants involving SH2B1 (157). Indeed, GWAS studies have suggested a role for common variants in SH2B1in human obesity (59). While the deletion of Sh2b1 from LepRb neurons in mice promotes obesity, this effect may be independent of leptin action (158), suggesting that SH2B1 impacts energy balance via its actions on other growth factor receptors.
Potential Roles for Other Adipokines and Anorexigenic Signals
Several lines of evidence suggest the existence of peripherally-derived anorexigenic signals in addition to leptin and insulin. First, because continuous administration of high levels of exogenous leptin in wild-type animals only slightly and transiently decreases feeding, while wild-type animals starve themselves to death during parabiosis to Leprdb/dbanimals (13,108,113,159), , there likely exists an additional hormonal signal that suppresses food intake (albeit one that requires leptin for its action). Additionally, the forced overfeeding of animals results in multi-day anorexia even in the absence of increased leptin concentrations (160). Although it is not clear that this second anorectic signal derives from adipose tissue, fat produces many signaling molecules in addition to leptin, some of which, like leptin, are cytokines (adipose-derived cytokines, or “adipokines”). While the adipokines adiponectin and resistin can alter feeding when injected into the brain (161,162), neither can suppress food intake to the extent observed in parabiosed or overfed animals. Thus, additional anorexigenic signals remain to be discovered.
The Orexigenic Ghrelin System
The diurnal release of ghrelin, which derives from the stomach, coincides with the initiation of meals and decreases over the course of each meal (163). Acutely administered ghrelin causes animals and humans to consume larger meals than normal, while chronic ghrelin administration results in obesity in rodents (164–167). As would be expected, most obese humans have low levels of circulating ghrelin, whereas levels are elevated in patients with anorexia nervosa (168).
The growth hormone secretagogue receptor (GHSR) serves as the receptor for the acylated (active) form of ghrelin (which is acylated (octanoylated) by ghrelin O-acyl transferase (GOAT) in the cells that synthesize it) (169). Ingested fatty acids are required for ghrelin acylation, so that active ghrelin only increases prior to meals in animals that have fed over the prior 24 hours.
ARC AgRP neurons express high levels of GHSR, and ghrelin activates these cells. Indeed, ghrelin action on AgRP neurons mediates the majority of the anorectic response to ghrelin (170,171). Consistent with the modest baseline phenotypes of mice null for the individual neurotransmitters employed by AgRP/NPY neurons, mice null for ghrelin, GHSR, or GOAT beginning early in embryogenesis exhibit no detectable alterations in baseline energy balance, and only modest defects in refeeding (172), presumably due to compensatory processes that alter the function of AgRP neurons during development. Apart from its actions on neurons in the ARC, ghrelin administration into other areas of the brain (i.e. PVN, LHA, ventral tegmental area (VTA), dorsal vagal complex) can also stimulate positive energy balance (173–176).
THE HINDBRAIN CONTROL OF FEEDING
Most consider the hypothalamus to play a dominant role in the long-term control of food intake. Indeed, leptin, the hormonal signal of long-term energy stores, mediates its largest effects on food intake and energy balance via the hypothalamus (122,177). In contrast, hindbrain circuits respond robustly to signals of gut status (including stretch, nutrients, and toxins/irritants) to control meal termination and thus meal size.
Humoral signals from the gut act on the hindbrain area postrema (AP), which lies outside the blood-brain barrier at the base of the fourth ventricle in the caudal medulla. Other gut signals are conveyed to the hindbrain via afferent vagal fibers (whose soma lie in the nodose ganglion) (Figure 4). These signals converge on the nucleus tractus solitarius(NTS) and promote meal termination (178,179). Interference with components of this system (e.g., vagotomy) increases meal size, although compensatory changes in meal frequency (presumably directed by the hypothalamus) often dictate that food intake and energy balance remain constant over the long-term (180). Outputs from the AP and NTS include the dorsal motor nucleus of the vagus (DMV), which sends parasympathetic signals to the gut to alter motility. Projections to more rostral regions, including the PBN and hypothalamic sites (including the PVH and DMH) also play roles in the suppression of food intake.
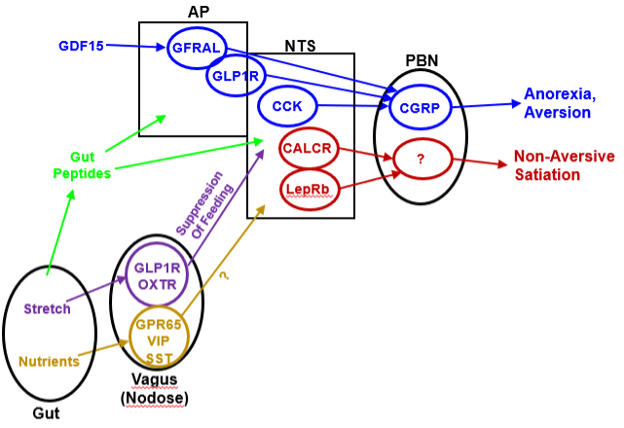
Figure 4. Emerging circuitry of gut-brain pathways that control food intake. A variety of signals converge on the hindbrain to suppress food intake. This includes a variety of gut peptides and the stress/inflammation signal, GDF15, as well as vagal sensory neurons whose soma reside in the nodose ganglion. Stretch-sensing vagal afferents that express GLP1R and/or OXTR suppress feeding via the NTS (although their particular cell targets in the NTS remain to be defined). In contrast, nutrient-sensing vagal neurons (including those that express GPR65, VIP, and/or SST) do not appear to control feeding; their precise function remains undefined. Many populations of AP/NTS neurons promote the aversive suppression of food intake by projecting onto CGRP-expressing cells of the PBN. Other neurons of the NTS (including those that express CALCR and LepRb) suppress food intake without promoting aversive effects, at least in part by activating a poorly-defined set of non-CGRP neurons in the PBN.
A number of observations suggest potential roles for hindbrain centers in the control of long-term energy balance, however, including the expression of LepRb and GHSR in the AP and NTS (83,84,181–184). Indeed, leptin modulates the physiology of hindbrain neurons and knockdown of NTS LepRb expression modestly increases food intake and body weight, especially in high fat diet (HFD)-fed rats (181,185–189). Furthermore, ablation of prolactin releasing hormone (PRLH, a.k.a., PRRP) increases feeding and body weight, and the NTS-specific re-expression of PRLH on a Prlh-null background restores normal feeding and energy balance (190). More recently, the silencing of several NTS cell types has been shown to increase food intake and cause obesity. Thus, the normal function of NTS systems contributes to the long-term control of energy balance. Furthermore, many appetite-suppressing medications (including agonists for gut peptide receptors) mediate their effects by activating hindbrain systems (191–194).
The Nodose Ganglion and Vagal Sensory Neurons
Gut-innervating vagal sensory neurons in the nodose ganglion consist of mechanosensory cells that increase activity in relation to increasing gastric volume and distinct chemosensory neurons that respond to the chemical characteristics of nutrients in the gut. Both mechanosensing and chemosensing vagal neurons innervate the entire gastrointestinal tract (195,196). Recent studies have interrogated the vagal sensory neurons of the nodose ganglion, revealing markers for gut-innervating mechanosensory cells (which sense stretch and pressure; these cells express the receptors for GLP1 (GLP1R) and OXT (OXTR)) and for chemosensory neurons (which sense nutrients in the gut; these cells express GPR65, vasoactive intestinal peptide (VIP), and somatostatin (SST)) (197,199). Interestingly, the activation of mechanosensory cells suppresses feeding, while chemosensory cell activation does not. Thus, the mechanosensory and chemosensory vagal cells must innervate distinct downstream CNS targets, at least in part. The appetite-suppressing functions of several hormones and neuropeptides (including gut-derived cholecystokinin (CCK)) may result from their actions on vagal neurons (200,201). While CNS OXT neurons (in the PVH) do not appear to participate the in the control of feeding, the response of vagal mechanosensory neurons to exogenous OXTR agonists might mediate the appetite-suppressing effects of these agents (202).
Role for the Area Postrema in Nausea and Aversive Responses
Because AP capillaries lack tight junctions, the AP lies outside the blood-brain barrier and directly senses circulating nutrients and hormones. While the molecular characterization of AP neurons remains in its infancy, the AP contains a variety of receptors (GLP1R, GFRAL, and CALCR) that respond to appetite-suppressing hormones (203–206). Notably, ligands for each of these receptors promote aversive responses (e.g., nausea), for which the AP is well-known (207–209). Indeed, the action of autoantibodies directed to aquaporin-4 (AQP4, which is expressed around the AP) during neuromyelitis optica spectrum disorders results in AP syndrome- characterized by unremitting nausea and vomiting (and sometimes hiccups) (210–212). Neurons from the AP project into the brain, including to the NTS, DMV, and PBN.
The Nucleus Tractus Solitarius and Parabrachial Nucleus
The NTS, which lies adjacent to the AP, receives gastrointestinal input from vagal sensory neurons and from the AP. The NTS also receives taste information via the geniculate ganglion (213), although the NTS systems that integrate taste signals with information from the gut have yet to be defined. NTS neurons also express a variety of receptors that contribute to the control of food intake (e.g., LepRb and CALCR), and thus presumably sense a variety of circulating appetite-regulating signals. Furthermore, NTS LepRb and CALCR neurons contribute to the physiologic control of food intake (185,214,215). Interestingly, while at least some AP and NTS neurons mediate the aversive suppression of food intake (i.e., cause nausea and/or vomiting, as well as decreasing appetite), the NTS LepRb and CALCR neurons suppress food intake without promoting such aversive responses (214,215).
Thus, distinct NTS systems mediate the aversive and non-aversive suppression of food intake. Indeed, it makes teleological sense that the consumption of nutrients should promote reward (to encourage the subsequent ingestion of a particular food type), rather than terminating ingestion in an aversive manner and discouraging the future consumption of the food. Consistently, the activation of certain vagal pathways can promote a rewarding response, even while suppressing feeding (198,199).
Many AP/NTS neurons that mediate the aversive suppression of food intake directly innervate calcitonin gene-related protein (CGRP)-expressing PBN neurons. Indeed, PBN CGRP neurons mediate the aversive responses to a variety of agents associated with gut irritation, including some chemotherapy drugs (216). PBN CGRP cells also appear to participate in the emotional response to a variety of fear-inducing stimuli (217). The activation of PBN CGRP cells suppresses food intake under a variety of conditions; indeed, the withdrawal of inhibitory tone from these cells mediates the lethal anorexia associated with the ablation of ARC AgRP neurons (65).
Interestingly, however, the inactivation of PBN CGRP cells minimally impacts food intake and does not alter energy balance (218); thus other neural systems must mediate the long-term control of feeding and energy balance by brainstem systems. Hence, the systems that mediate the aversive suppression of food intake may suppress long-term feeding less effectively than non-aversive systems, at least under normal physiologic conditions. The PBN must also contain non-aversive systems for the suppression of food intake, since neither NTS CALCR cells nor PVH MC4R neurons innervate PBN CGRP cells (but rather innervate a distinct region of the PBN) and both promote the non-aversive suppression of food intake via the PBN (214).
Gastrointestinal Hormones that Modulate Feeding
CHOLECYSTOKININ
Secreted from neuroendocrine secretory cells (L-cells) lining the intestinal lumen in response to nutrients, cholecystokinin (CCK) represents the canonical gut-derived satiety signal. It is an acutely acting signal with a very short half-life (219). Early studies showed that exogenous CCK administered just prior to a meal reduces food intake in rats. In the last thirty years these results have been repeated and extended in numerous labs, demonstrating that the anorectic effects of CCK can be translated to virtually all species, including humans (220–222). CCK induces a transitory sensation of satiety, secretion of pancreatic enzymes and gallbladder contraction. CCK-A receptors are located on vagal afferents of the stomach and the liver and transduce signals via the vagal nerve to satiety centers in the brainstem, eliciting a brief reduction in food intake (for a review, see(Bray 2000) (223)). While CCK decreases meal size and duration, compensatory increases in meal frequency prevent CCK from producing long term effects on total food intake or body weight. Indeed, deletion of Cckar in mice does not cause obesity (224).
THE INCRETINS
Glucagon like peptide-1 (GLP-1) functions as an incretin (enhancer of insulin secretion) (225). GLP-1 can also modulate satiety: ICV GLP-1 (or GLP1R agonists) potently suppresses food intake in rats and mice, while the GLP1R antagonist, exendin (9-37), increases short-term food intake. Body weight and food intake are unaffected by ablation of GLP-1R, however, suggesting that (like CCK and CCKAR) this system primarily modulates short-term satiation, rather than long-term energy balance, under normal physiologic circumstances (226). Despite this lack of a physiological role for GLP-1 or GLP-1R in the long-term control of food intake, chronic treatment with GLP-1R agonists serves to suppress food intake and promote weight loss (227).
The suppression of food intake by GLP-1R agonist pharmacotherapy requires GLP-1R expression on glutamatergic neurons of the CNS (194). Furthermore, caudal brainstem processing suffices to suppress food intake and gastric emptying by peripherally applied GLP-1R agonists (228). Thus, the crucial GLP-1R-expressing neurons that mediate the anorectic effects of GLP-1R agonist pharmacotherapy may reside in the AP and/or NTS.
Given that brain GLP-1R mediates the appetite-suppressing effects of exogenous GLP-1R agonists and that the NTS GLP-1 neurons represent the sole source of GLP-1 in the CNS (229), these NTS GLP-1 cells have been the subject of a great deal of interest. Interestingly, however, while NTS GLP-1 cells represent a subset of the NTS LepRb cells that contribute to the control of feeding, the ablation of NTS GLP-1 fails to alter energy balance or the ability of NTS LepRb neurons to suppress feeding (215). Consistently, extending the half-life of endogenous GLP-1 by inhibiting dipeptidylpetidase-4 (DPP4) fails to alter food intake, although it amplifies the incretin effect of endogenous GLP-1. Thus, neither endogenous NTS GLP-1 nor its CNS targets contribute meaningfully to the suppression of food intake, despite the prominent pharmacologic effects of GLP-1R agonists on these parameters.
Intestinal glucose-dependent insulinotropic polypeptide (GIP, formerly gastric inhibitory polypeptide) is secreted from K-cells in the duodenum and proximal jejunum in response to food intake (230,231) and acts as an incretin, increasing glucose-dependent insulin release from pancreatic β-cells and contributing to postprandial plasma glucose normalization. The incretin function of GIP may be mediated either directly via pancreatic GIP receptor (GIPR) activation (232) or via the activation of non-ganglionic cholinergic neurons that innervate the islets, presumably as part of an enteric-neuronal-pancreatic pathway (233). The impact of GIP on central appetite regulation is controversial, however (234,235). Indeed, while the combination of GIPR and GLP1R agonism in a single peptide appears to enhance weight loss over a GLP1R agonist alone, GIPR ligands poorly modulate food intake on their own. Furthermore, there remains some debate about whether GIPR antagonism (rather than agonism) accentuates the effects of GLP1R agonists on food intake (236).
GROWTH DIFFERENTION FACTOR-15
While not a gut-derived peptide, growth differentiation factor 15 (GDF15) acts via the brainstem to modulate nutrient intake. GDF15 is secreted by a large number of tissues in response to cellular stressors. Circulating concentrations of GDF15 express increase in disease states, such as prostate cancer, infection, and cardiovascular disease, and this has been associated with anorexia and cancer cachexia (237). Furthermore, a variety of clinical and genetic data suggest roles for high circulating levels of GDF15 in the nausea and vomiting associated with hyperemesis gravidarum during the second trimester of pregnancy (238,239). Mice with transgenic over-expression of GDF15 are leaner and are protected from diet induced obesity, and the injection of GDF15 causes hypophagia and weight loss in rodents (240,241).
Unlike GDF15, which has broad tissue expression, expression of the receptor for GDF15 (GFRAL) is restricted to the AP and NTS in adults. Intact signaling through the hindbrain is required for GDF15-mediated weight loss, as ablation of the AP and NTS or deletion of GFRAL abolishes hypophagia and weight loss in GDF15-treated mice (205,242,243). While GDF15 produces a strong conditioned taste aversion, the downstream neural circuits by which GDF15/GFRAL activation modulates feeding behavior have yet to be elucidated. While GFRAL-null mice are protected from weight loss in response to infections, tumors, and chemotherapy, they display little (if any) alteration in body weight under normal physiologic conditions (204). Thus, GDF15 appears to link strong physiologic stressors (e.g., infection, pregnancy, cancer, and cardiovascular dysfunction) to the aversive suppression of food intake, rather than contributing to the normal control of food intake and energy balance.
PEPTIDE YY
Peptide YY (PYY), which is released from the L cells of the distal digestive tract, belongs to the pancreatic polypeptide family (including pancreatic polypeptide (PP) and NPY) and has been proposed to serve as a satiety signal (244–246). The circulation contains two forms of the peptide: PYY1-36 and PYY3-36; the latter represents the main circulating form of PYY in postprandial human plasma and is able to cross the blood-brain-barrier by non-saturable mechanisms (247,248). Both forms of PYY bind to the Y2 isoform of the NPY receptor (NPY2R) (249). While the reported effects of PYY3-36 on food intake in rodents and humans initially generated some controversy (250), recent studies support the notion that NPY2R agonists can promote a strongly aversive suppression of food intake in many species (251,252). The role for endogenous PYY in food intake remains unclear, however, and although the AP/NTS represent presumptive sites that mediate the suppression of food intake by NPY2R agonists, this has yet to be definitively established.
[Please refer to ENDOTEXT chapter Endocrinology of the Gut and the Regulation of Body Weight and Metabolism byAndrea Pucci and Rachel L Batterham, for additional information]
AMYLIN
Pancreatic b-cells co-secrete the peptide, amylin, with insulin during meals. Amylin inhibits gastric emptying and systemic and central administration causes a dose-dependent reduction of meal size (253–256). Amylin binds to the amylin receptor- CALCR in complex with a receptor activity modifying protein (RAMP) (257). The amylin-responsive neurons of the AP/NTS have yet to be definitively identified, but may lie in the AP and/or NTS. Interestingly, combination treatment with amylin plus leptin elicits a greater inhibition of food intake and body weight loss in obese rats than predicted by the sum of monotherapy conditions. Peripheral administration of amylin restores leptin sensitivity in rats, crucial in the treatment of leptin resistance in obesity (258), suggesting the potential therapeutic utility of combining hindbrain- and hypothalamus-acting compounds.
Interactions Between Forebrain and Brainstem Systems that Control Food Intake
Communication between the systems that sense the gut and those that sense energy stores is crucial to control satiety appropriately for feeding state and physiologic requirements. Thus, the forebrain and hindbrain must communicate to appropriately control feeding. Indeed, hypothalamic systems impact brainstem feeding circuits: AgRP neurons tonically inhibit PBN CGRP cells, while PVH projections to distinct (non-CGRP) PBN cells suppress feeding (61,65,70,71). Similarly, the ingestion of nutrients activates a gut-vagus-NTS pathway that inhibits the activity of AgRP neurons (199), and projections from the NTS to the PVH can blunt food intake (259). A great deal more research in this area will be required to fully understand the integration of these circuits, however.
OTHER SIGNALS THAT MAY MODULATE FOOD INTAKE
Nutrient Signaling
While their effects are not as strong as those of many hormones or neural circuits, all three groups of nutrients (carbohydrates, lipids, and proteins) have been implicated in the control of feeding. Mayer proposed the “glucostatic hypothesis” in the 1950s, suggesting that decreases in glucose utilization stimulated eating and increases in glucose utilization halted eating (260,261). Indeed, intrahypothalamic glucose administration decreases food intake and inhibits hepatic glucose production (262). The response to decreased glucose or the blockade of glycolysis, which increases food intake and hepatic glucose production, is much stronger than the response to increased glucose, however. Furthermore, most glucose-sensing neurons are modulated within the normal to low range of glucose concentrations, rather than by elevated glucose. Also, the sensor of cellular energy deficits, AMPK, has also been proposed to play a role in CNS glucose sensing (263,264), but this cellular pathway is likely to be engaged mainly by severe energy deficits in the CNS. Hence, the brain glucose- and energy-sensing systems may be mainly involved in defending against large swings in blood glucose (e.g., defending against hypoglycemia) rather than serving as a primary controller of food intake and energy balance.
While the hypothalamic sensing of long-chain fatty acids has also been suggested to suppress food intake in response to increased availability of fatty acids in states of nutrient surfeit (265,266), the physiologic relevance of such a system remains unclear. The uptake of esterified lipids into the CNS is modest and circulating fatty acids actually increase during fasting. The systems that import fatty acyl-CoAs into mitochondria and the control of overall mitochondrial function in hypothalamic cells that control food intake and metabolism represent important determinants of energy balance, however.
Low protein diets dramatically increase food intake, and the peripheral or intra-CNS infusion of amino acids (especially the branched-chain amino acid leucine) robustly decreases food intake (267,268). While the neural pathways underlying these effects have yet to be completely elucidated, brainstem systems likely contribute, at least in part. Additionally, the mechanistic target of rapamycin (mTOR)-mediated cellular amino acid sensing system is required for the operation of the CNS systems that mediate protein appetite (269). In addition to its role in neurotransmission, glutamate acts on its receptor in the GI tract both mediate taste-sensation and to serve as a gut-derived signal to also the vagal input to the CNS (270). In one study, intra-luminal glutamate infusion resulted in reduced body weight without altering food intake (271).
Inflammation
Inflammatory signals are proposed to mediate several distinct metabolic responses. Strong acute inflammatory stimuli (including those associated with systemic infection, cancer, etc.) decrease appetite and increase energy expenditure, promoting cachexia (GDF15 may mediate a portion of this effect). Conversely, obesity is associated with increased low-grade inflammation that appears limited to particular tissues, such as adipose tissue (272). This low-grade “metabolic inflammation” is associated with insulin resistance and obesity. A variety of animal models have been employed to explore the interaction of inflammatory signals and energy balance/metabolism.
SYSTEMIC INFLAMMATION
Systemic immune signaling promotes negative energy balance. Lipopolysaccharide (LPS) administration, which produces some of the metabolic consequences of bacterial infection, blunts appetite; the mechanism of this hypophagia overlaps with the systems that control energy balance, as the LPS-induced anorexia requires the melanocortin system (273). Consistent with the induction of negative energy balance by systemic inflammation, alterations that blunt inflammation generally blunt inflammatory anorexia. While not altering baseline energy balance in chow-fed animals, deletion of IL-1b converting enzyme (ICE, which is essential for IL-1b activity), prevents LPS-induced anorexia in mice (274). GDF15, acting via AP GFRAL neurons, may also contribute to the LPS-mediated suppression of food intake.
The inflammatory system may also contribute to the control of energy balance under normal physiology, as well: adiposity is increased in Il6 null and Gmcsf null mice, and in mice with impaired macrophage function due to the targeted deletion of Mac-1 or LFA-1 (or their receptor, ICAM-1)(275). Conversely, mice with constitutively increased IL-1 receptor signaling induced by targeted deletion of the endogenous IL-1 receptor antagonist, Il1ra, display reduced body mass compared to wild-type littermates (276).
METABOLIC INFLAMMATION
Obesity is associated with increased production of a number of cytokines (including TNF alpha) in adipose tissue, resulting primarily from the activation of adipose tissue macrophages and other immune cells (275,279). Manipulations that decrease adipose tissue inflammation ameliorate the metabolic dysfunction associated with obesity. While interference with generalized macrophage function may increase adiposity, interventions that alter their pro-inflammatory (versus anti-inflammatory) nature increase leanness and improve metabolic function (280,281).
Some data also suggest that inflammation-associated hypothalamic processes may contribute to obesity. High fat feeding results in the activation of hypothalamic microglia (the resident immune cells of the brain) and astrocytes (282,283). Some have postulated that these activated microglia secrete proinflammatory cytokines to disrupt the control of food intake, promoting obesity. Debate continues regarding whether this gliosis provokes or attenuates obesity, however. The ER stress in adipose tissue and the hypothalamus, potentially a consequence of metabolic inflammation, has also been reported in obesity (284). Genetic or pharmacologic interference with ER stress ameliorates obesity and insulin resistance in rodent models.
ENERGY BALANCE AND MOTIVATION
The homeostatic regulation of energy balance powerfully defends against body weight excursions below the lower limits of adiposity (9), and but often fails to prevent weight gain in our world of abundance of highly palatable, high energy foods. Non-metabolic factors that contribute to overeating and obesity include food palatability, availability, sensory-specific satiety, energy density of food, consumption rate, stress, social environment and energy output/exercise (285,286). Palatability and pleasantness of food represent powerful determinants in regulating motivation to eat.
Reward Circuitry and Neurotransmitters
DOPAMINE AND THE BRAIN REWARD SYSTEM
The neural circuits that comprise the reward pathways encompass wide-ranging brain regions, including the hypothalamus, the nucleus acumbens in the basal forebrain, the midbrain ventral tegmental area (VTA), the amygdala and the thalamus (274). The LHA connects the hypothalamus to the broader reward system through projections to the VTA, where dopaminergic cell bodies lie. From there, the mesolimbic pathways (dopaminergic projections between the VTA and the nucleus acumbens) mediate reward-based feeding (287–289).
Dopamine (DA) potently augments the drive to obtain a rewarding stimulus and is required to drive feeding behavior. DA-deficient mice nurse normally until 2 weeks of age, but thereafter fail to thrive due an inability to wean themselves onto solid food unless supplemented with the DA precursor, L-DOPA, suggesting that DA is required for normal ingestive behavior (as well as activity) (290). While the specific mechanisms through which dopaminergic signaling regulates motivated feeding behavior are not yet clear, connections between the LHA and the mesolimbic system as well as integration with the leptin and melanocortin systems appear to contribute.
SEROTONIN RECEPTOR 2c
Serotonin (5-hydroxytrypamine, 5-HT), which derives from stress-modulated neurons in the midbrain raphe nuclei, acts via 5-HT receptor 2c (HTR2c) to decrease food intake and body weight, and deletion of Htr2c produces hyperphagic obesity that is accentuated by high fat diet. Within the hypothalamus, ARC, PVN, LHA, and anterior hypothalamic nucleus (AH) neurons contain Htr2c (291). A subset of ARC POMC neurons express Htr2c, and the Pomccre-mediated reactivation of a null Htr2c allele in these cells attenuates the food intake and obesity in the Htr2cnull mice (292,293). Htr2c cells in the midbrain VTA and in the hindbrain NTS may also contribute to the control of feeding by HTR2c. The effect of HTR2c activation may vary by brain region, but, in aggregate, Htr2c mutant mice confirm the important role for this receptor in energy balance. HTR2c agonists promote weight loss, and several have been approved for the treatment of obesity.
ENERGY EXPENDITURE AS A DETERMINANT OF ADIPOSITY
With few exceptions, most of the systems that dramatically alter energy balance act primarily via the control of feeding; isolated alterations in energy expenditure promote more modest changes in energy balance because increases in energy expenditure and negative energy balance promote a compensatory increase in feeding. Similarly, decreased energy expenditure will cause the accumulation of adipose mass, which tends to restrain feeding. For instance, interference with normal VMH function (discussed above) decreases diet-induced energy expenditure and promotes increased adiposity only when animals are provided high caloric density diets (91,92).
The tendency for energy intake to match changes in energy expenditure is exemplified by several animal models in which alterations in energy expenditure do not lead to large changes in adiposity. Uncoupling protein 1 (UCP1, which is found primarily in brown and beige adipose tissue (BAT)) allows dissipation of the electrochemical gradient across the inner mitochondrial membrane, releasing energy as heat (294). Ablation of BAT in mice expressing diphtheria toxin A driven from the UCP1 promoter or congenital deletion of Ucp1 fails to alter adiposity at thermoneutrality, although adiposity increases slightly relative to controls in animals raised at temperatures colder than thermoneutrality, since these animals fail to substantially increase energy expenditure in response to the cold challenge (295). Similarly, the phenotype of mice null for the beta-adrenergic receptor beta 3-AR is not as severe as predicted: fat mass in male mice is only slightly increased, even in animals consuming a high-energy diet under non-thermoneutral conditions (296). Also, “beta-less” mice, with a global targeted deletion of all three beta-adrenergic receptor isoforms, have only slightly increased body fat on high fat diet under non-thermoneutral conditions (296).
[Please refer to ENDOTEXT chapter titled The Role of Non-exercise Activity Thermogenesis in Human Obesity byChristian von Loeffelholz and Andreas Birkenfeld and Control of Energy Expenditure in Humans by Klaas R Westerterp for additional complementary information on energy expenditure]
LESSONS FROM HUMAN OBESITY SYNDROMES
While much of our understanding of the genetics and signaling pathways involved in the central control of energy balance and development of obesity has been derived from rodent models, there exist rare cases of human obesity syndromes due to genetic mutations that shed light on the pathogenesis of obesity development. Many of these mutations corroborate the evidence from animal studies. In addition, with the advent of next generation sequencing and the ability to delve deeply into the human genome, genome wide association studies (GWAS) have also begun to reveal gene variants that may contribute or predispose to obesity.
Monogenic Obesity Syndromes
MC4R
Approximately 4% of morbid human obesity (BMI > 40 kg/m2) results from mutations in MC4R (297–299). Preserved lean mass and increased stature are also evident in humans with MC4R deficiency syndrome, as in rodent models (57). Most obesity associated with MC4R mutations has been attributed to heterozygosity at the MC4R locus (58). Patients who are homozygous for a null MC4R mutation develop severe childhood obesity (57), while heterozygous family members are overweight. This suggests a codominant inheritance pattern in which the gene product of these mutations impair the function of the normal gene product. Genome-wide association studies (GWAS) have revealed common non-coding polymorphisms within the MC4R locus that are associated with increased adiposity (59). Treatment options for patients with MC4R mutations remain limited, although recent studies have suggested that the newly developed MC4R agonist setmelanotide can produce modest weight loss in patients with MC4R variants that encode receptor with decreased (rather than absent) function, as well as those with POMC mutations (300,301).
LEPTIN DEFICIENCY INCLUDING LIPODYSTROPHY
Genetic leptin deficiency in humans is very rare, but (as in rodents) elicits a severe obesity phenotype: A rare, recessively inherited LEP mutation was discovered in two children who are members of a highly consanguineous Pakistani family (302). This frameshift mutation introduces a premature stop codon that truncates the leptin protein. While rare, additional leptin-deficient individuals (all of whom are severely obese) have been identified. Daily subcutaneous administration of recombinant leptin dramatically and selectively reduces body fat to normal levels in these individuals (303). A few humans homozygous for leptin receptor mutations have also been identified; these individuals present a severe obese phenotype similar to those lacking leptin, although – as anticipated - they are not responsive to exogenous leptin (304). It is important to note that mice (305) and humans (306) heterozygous for null mutations of either LEPR or LEP are more obese than controls. It is thus possible that individuals heterozygous for functionally null mutations of these and other genes encoding molecular components of the various signaling pathways regulating energy homeostasis discussed in this review constitute a significant proportion of the very obese. Additionally, heterozygosity for several of these mutations would be expected to produce even greater levels of obesity. The increasing use of exome sequencing in evaluating instances of severe obesity will lead to the detection of more instances of obesity caused by such oligogenic mechanisms.
Lipodystrophy represents another clinical syndrome associated with leptin deficiency. Lipodystrophy encompasses a heterogenous group of disorders that range from inherited monogenic gene disruptions to acquired disorders due to treatment with medications such as highly active retroviral therapy for HIV. In generalized lipodystrophy, patients develop loss of subcutaneous fat tissue which results in leptin deficiency, hyperphagia, severe insulin resistance and diabetes, and visceral obesity. When leptin deficiency can be demonstrated, treatment with recombinant leptin significantly improves hyperphagia, body weight and diabetes severity (307).
CILIOPATHIES
A subset of mutations causing defects in primary cilia promote obesity syndromes (308,309). The primary cilium is found on most cells; while structurally related to motile cilia (such as flagella), the primary cilium is immotile and does not participate in propulsion. The primary cilium plays a crucial sensory role in cells, including cell-specific sensing, such as olfaction in sensory epithelium, photoreception in retinal cells, mechanical transduction in kidney cells, and signaling via a variety of cell surface receptors, including many GPCRs. A broad group of disease-causing human mutations are now known to result from mutations in genes affecting ciliary functions (the “ciliopathies”). The clinical presentation of these diseases variably includes anosmia, retinal degeneration, kidney malformations, and a variety of developmental and neural defects, many of which are idiosyncratic to the particular gene that is mutated. A number of these mutations produce obesity in addition to the other phenotypes noted above, both in mice and humans. Included in these obesity-causing ciliopathies are Bardet-Biedel Syndrome (BBS), McKusic-Kaufman Syndrome, Alström Syndrome, and Joubert Syndrome. Altered trafficking of MC4R and/or MCH receptor may play roles in the obesity of those with ciliopathies.
POMC AND PROHORMONE CONVERTASE 1 DEFICIENCIES
Mutations resulting in complete absence of the POMC gene product cause secondary adrenal insufficiency due to lack of ACTH; however, once glucocorticoid replacement therapy is started, children with these mutations invariably develop obesity due to hyperphagia. In patients of Caucasian ancestry, there is also a characteristic phenotype of red hair and pale skin, although this is not found in patients from other genetic backgrounds. Some POMC mutations affect specific melanocortin peptide products, and those that specifically alter α-MSH result in severe early-onset obesity (39,40).
The prohormone POMC is cleaved by prohormone convertase 1 (PC1). Human PC1 deficiency caused by missense and splice site mutations in the PC1 gene also results in a disorder characterized by obesity and hypocortisolemia as well as hypogonadism (310). Other monogenetic obesity syndromes in mice and humans likely result from alterations in melanocortin signaling, including those due to alterations in other components of the peptide processing system, including carboxypeptidase E (CPE) (311).
BRAIN-DERIVED NEUROTROPHIS FACTOR (BDNF/TrkB) SIGNALING
BDNF, a member of the neurotrophin family, is widely expressed in the nervous system during development, as well as being expressed within several brain regions important for energy homeostasis in adults (312). BDNF acts via its receptor, TrkB, to control a variety of basic neural processes, including proliferation, survival, and plasticity. Given its many important roles in the CNS, alterations in BDNF (or its receptor, TrkB) would be predicted to interfere with multiple processes. Indeed, humans haploinsufficient for BDNF display impaired cognitive function and hyperactivity, in addition to hyperphagic obesity (313,314). Mutations in NTRK2 (which encodes TrkB) produce similar hyperphagia and obesity, along with impaired cognitive function and nociception, in rare human patients (315). Interestingly, a coding polymorphism in BDNF (Val66Met) is associated both with obesity and with binge eating disorders in humans (316), consistent with the role for BDNF/TrkB signaling in energy balance, and suggesting a broader role for this system in the genetic determination of adiposity in humans. Indeed, alteration of TrkB and/or BDNF function in the hypothalamus of mice promotes obesity (317,318). Furthermore, polymorphisms in BDNF are associated with risk for obesity in human GWAS studies (59).
PRADER-WILLI SYNDROME (PWS)
PWS presents in infancy with low birth weight, hypotonia and poor feeding, followed by a progressive transition to hyperphagia and obesity starting after age 2 or 3 years. Additional features include short stature (correctible with growth hormone therapy), central hypogonadism, characteristic behaviors (especially around feeding), and often cognitive impairment (319,320). Most instances result from a 5-7 Mb deletion of an imprinted region (PWS region) on the paternal chromosome 15 (15q11-q13) and are non-recurrent. Within this deletion lie a number of genetic elements, including the genes encoding MAGEL2 and NECDIN, which are thought to be involved in neural development and function, and a complex non-coding locus. Non protein -coding genes in this interval include a transcribed non-coding gene (SNURF-SNRPN) that encodes a multitude of C/D box small nucleolar (sno-) RNA genes, including SNORD116. The RNA products of these SNORD genes are thought to be involved in RNA editing, perhaps of specific mRNA species.
A small number of individuals with PWS phenotypes associated with microdeletions of the implicated region on chromosome 15 have reduced the number of candidate genes for this syndrome (319). These patients have demonstrated obesity, developmental delay, hypogonadism, and all major features of PWS. The minimum critical deletion region contains only non-coding genes, including the SNORD116 gene cluster, IPW, and SNORD109A. The Snord116 locus has been deleted from mouse models, which display a growth defect and behavioral abnormalities, including a relative hyperphagia that develops after weaning, but which is balanced by increased energy expenditure (321). Thus, the effects of SNORD116 likely contribute to PWS, but may not account for all of the phenotypes.
The functions of Necdin and Magel2 have also been examined in genetically targeted mouse models. Magel2-/- mice display early growth retardation with a mild increase in adiposity, and Necdin-/- mice display early postnatal respiratory failure along with a subset of PWS-associated behaviors (322–324). Thus, the full PWS likely results from the combined effects of multiple genes; several genes within the PWS region also likely contribute to the maximal obesity phenotype. It is not yet clear how each of the loci within the PWS alter neurophysiology and/or which neurons they might specifically affect to alter energy balance. Understanding the molecular physiology of PWS is likely to identify novel genes in the control of energy homeostasis in non-syndromic obesities.
[Please refer to the chapter titled The Genetics of Obesity in Humans by Sadaf Farooqi and Stephen O'Rahilly, for additional information on genetic forms of obesity.
CONCLUSION
This chapter provides an overview of the complex neural and metabolic pathways that determine energy intake and expenditure. Distinct areas of the brain receive and interpret hormonal and neuronal messages from the periphery and other brain regions to integrate regulatory changes that maintain energy balance. These changes require a finely tuned balance of synaptic neurotransmitters, hormonal feedback loops and neuropeptide expression. The identification of the molecules encoding these messages using human studies and animal models has expedited the discovery of the crucial signaling and homeostatic pathways that govern these mechanisms. Their existence provides definitive refutation of vitalist/psychological notions that have permeated the field of energy intake and metabolism, and provides the heuristic, reductionist framework in which ongoing research on these questions should be conducted. It is likely that major genes and their modifiers, as well as allelic variants of a larger number of genes with lesser individual impact, will eventually account for both qualitative and quantitative aspects of the critical phenotypes in rodents and humans.
REFERENCES
- Leibel RL. Energy In, Energy Out, and the Effects of Obesity-Related Genes. N Engl J Med. 2008 Dec 11;359(24):2603–4.
- Cahill GF. Physiology of Insulin In Man: The Banting Memorial Lecture 1971. Diabetes. 1971 Dec 1;20(12):785–99.
- Spiegelman BM, Flier JS. Obesity and the Regulation of Energy Balance. Cell. 2001 Feb;104(4):531–43.
- Bruch H. THE FRÖHLICH SYNDROME: REPORT OF THE ORIGINAL CASE. Am J Dis Child. 1939 Dec 1;58(6):1282.
- Hetherington AW, Ranson SW. Hypothalamic lesions and adiposity in the rat. Anat Rec. 1940 Oct;78(2):149–72.
- Stellar E. The physiology of motivation. Psychological Review. 1954;61(1):5–22.
- Harris RBS. Role of set‐point theory in regulation of body weight. FASEB j. 1990 Dec;4(15):3310–8.
- Hervey GR. The effects of lesions in the hypothalamus in parabiotic rats. The Journal of Physiology. 1959 Mar 3;145(2):336–52.
- Kennedy GC. The role of depot fat in the hypothalamic control of food intake in the rat. Proc R Soc Lond B Biol Sci. 1953 Jan 15;140(901):578–96.
- Ingalls AM, Dickie MM, Snell GD. OBESE, A NEW MUTATION IN THE HOUSE MOUSE*. Journal of Heredity. 1950 Dec;41(12):317–8.
- Hummel KP, Dickie MM, Coleman DL. Diabetes, a New Mutafton in the Mouse. Science. 1966 Sep 2;153(3740):1127–8.
- A hypothetical mechanism for the regulation of food intake in relation to energy balance. By G. R. Hervey, Department of Physiology, School of Medicine, University of Leeds. Proc Nutr Soc. 1969 Sep;28(2):54a–5a.
- Coleman DL. Effects of parabiosis of obese with diabetes and normal mice. Diabetologia. 1973 Aug;9(4):294–8.
- Coleman DL, Hummel KP. The effects of hypothalamics lesions in genetically diabetic mice. Diabetologia. 1970 Jun;6(3):263–7.
- Zhang Y, Proenca R, Maffei M, Barone M, Leopold L, Friedman JM. Positional cloning of the mouse obese gene and its human homologue. Nature. 1994 Dec;372(6505):425–32.
- Chua SC, White DW, Wu-Peng XS, Liu S-M, Okada N, Kershaw EE, et al. Phenotype of fatty Due to Gln269Pro Mutation in the Leptin Receptor (Lepr). Diabetes. 1996 Aug 1;45(8):1141–3.
- Chen H, Charlat O, Tartaglia LA, Woolf EA, Weng X, Ellis SJ, et al. Evidence That the Diabetes Gene Encodes the Leptin Receptor: Identification of a Mutation in the Leptin Receptor Gene in db/db Mice. Cell. 1996 Feb;84(3):491–5.
- Lee G-H, Proenca R, Montez JM, Carroll KM, Darvishzadeh JG, Lee JI, et al. Abnormal splicing of the leptin receptor in diabetic mice. Nature. 1996 Feb;379(6566):632–5.
- Kowalski TJ, Liu S-M, Leibel RL, Chua SC. Transgenic Complementation of Leptin-Receptor Deficiency: I. Rescue of the Obesity/Diabetes Phenotype of LEPR-Null Mice Expressing a LEPR-B Transgene. Diabetes. 2001 Feb 1;50(2):425–35.
- Fry M, Ferguson AV. The sensory circumventricular organs: Brain targets for circulating signals controlling ingestive behavior. Physiology & Behavior. 2007 Jul;91(4):413–23.
- Gold RM. Hypothalamic Obesity: The Myth of the Ventromedial Nucleus. Science. 1973 Nov 2;182(4111):488–90.
- Cone RD. Anatomy and regulation of the central melanocortin system. Nat Neurosci. 2005 May;8(5):571–8.
- Cuenot LCMJ. Les races pures et leurs combinaisons chez les Souris (Notes et Revue). Archives de Zoologie Experimentale [Internet]. 1905 [cited 2020 Nov 17];3(7). Available from: https://repository.cshl.edu/id/eprint/26595/
- Dickie MM. Mutations at the Agouti Locus in the Mouse. Journal of Heredity. 1969 Jan;60(1):20–5.
- Barsh GS, He L, Gunn TM. GENETIC AND BIOCHEMICAL STUDIES OF THE AGOUTI–ATTRACTIN SYSTEM. Journal of Receptors and Signal Transduction. 2002 Jan;22(1–4):63–77.
- Miller MW, Duhl DM, Vrieling H, Cordes SP, Ollmann MM, Winkes BM, et al. Cloning of the mouse agouti gene predicts a secreted protein ubiquitously expressed in mice carrying the lethal yellow mutation. Genes & Development. 1993 Mar 1;7(3):454–67.
- Duhl DMJ, Vrieling H, Miller KA, Wolff GL, Barsh GS. Neomorphic agouti mutations in obese yellow mice. Nat Genet. 1994 Sep;8(1):59–65.
- Cone RD, Lu D, Koppula S, Vage DI, Klungland H, Boston B, et al. The melanocortin receptors: agonists, antagonists, and the hormonal control of pigmentation. Recent Prog Horm Res. 1996;51:287–317; discussion 318.
- McMinn JE, Wilkinson CW, Havel PJ, Woods SC, Schwartz MW. Effect of intracerebroventricular α-MSH on food intake, adiposity, c-Fos induction, and neuropeptide expression. American Journal of Physiology-Regulatory, Integrative and Comparative Physiology. 2000 Aug 1;279(2):R695–703.
- Havel PJ. Peripheral Signals Conveying Metabolic Information to the Brain: Short-Term and Long-Term Regulation of Food Intake and Energy Homeostasis. Exp Biol Med (Maywood). 2001 Dec;226(11):963–77.
- Ollmann MM, Wilson BD, Yang YK, Kerns JA, Chen Y, Gantz I, et al. Antagonism of central melanocortin receptors in vitro and in vivo by agouti-related protein. Science. 1997 Oct 3;278(5335):135–8.
- Schwartz MW, Seeley RJ, Woods SC, Weigle DS, Campfield LA, Burn P, et al. Leptin Increases Hypothalamic Pro-opiomelanocortin mRNA Expression in the Rostral Arcuate Nucleus. Diabetes. 1997 Dec;46(12):2119–23.
- Wei Q, Krolewski DM, Moore S, Kumar V, Li F, Martin B, et al. Uneven balance of power between hypothalamic peptidergic neurons in the control of feeding. Proc Natl Acad Sci USA. 2018 Oct 2;115(40):E9489–98.
- Zhan C, Zhou J, Feng Q, Zhang J -e., Lin S, Bao J, et al. Acute and Long-Term Suppression of Feeding Behavior by POMC Neurons in the Brainstem and Hypothalamus, Respectively. Journal of Neuroscience. 2013 Feb 20;33(8):3624–32.
- Hentges ST. GABA Release from Proopiomelanocortin Neurons. Journal of Neuroscience. 2004 Feb 18;24(7):1578–83.
- Hentges ST, Otero-Corchon V, Pennock RL, King CM, Low MJ. Proopiomelanocortin Expression in both GABA and Glutamate Neurons. Journal of Neuroscience. 2009 Oct 28;29(43):13684–90.
- Anderson EJP, Çakir I, Carrington SJ, Cone RD, Ghamari-Langroudi M, Gillyard T, et al. 60 YEARS OF POMC: Regulation of feeding and energy homeostasis by α-MSH. Journal of Molecular Endocrinology. 2016 May;56(4):T157–74.
- Bumaschny VF, Yamashita M, Casas-Cordero R, Otero-Corchón V, de Souza FSJ, Rubinstein M, et al. Obesity-programmed mice are rescued by early genetic intervention. J Clin Invest. 2012 Nov;122(11):4203–12.
- Krude H, Biebermann H, Luck W, Horn R, Brabant G, Grüters A. Severe early-onset obesity, adrenal insufficiency and red hair pigmentation caused by POMC mutations in humans. Nat Genet. 1998 Jun;19(2):155–7.
- Krude H, Biebermann H, Schnabel D, Tansek MZ, Theunissen P, Mullis PE, et al. Obesity due to proopiomelanocortin deficiency: three new cases and treatment trials with thyroid hormone and ACTH4-10. J Clin Endocrinol Metab. 2003 Oct;88(10):4633–40.
- Farooqi IS, Drop S, Clements A, Keogh JM, Biernacka J, Lowenbein S, et al. Heterozygosity for a POMC-null mutation and increased obesity risk in humans. Diabetes. 2006 Sep;55(9):2549–53.
- Ramachandrappa S, Farooqi IS. Genetic approaches to understanding human obesity. J Clin Invest. 2011 Jun;121(6):2080–6.
- Luquet S, Perez FA, Hnasko TS, Palmiter RD. NPY/AgRP neurons are essential for feeding in adult mice but can be ablated in neonates. Science. 2005 Oct 28;310(5748):683–5.
- Gropp E, Shanabrough M, Borok E, Xu AW, Janoschek R, Buch T, et al. Agouti-related peptide-expressing neurons are mandatory for feeding. Nat Neurosci. 2005 Oct;8(10):1289–91.
- Qian S, Chen H, Weingarth D, Trumbauer ME, Novi DE, Guan X, et al. Neither agouti-related protein nor neuropeptide Y is critically required for the regulation of energy homeostasis in mice. Mol Cell Biol. 2002 Jul;22(14):5027–35.
- Tong Q, Ye C-P, Jones JE, Elmquist JK, Lowell BB. Synaptic release of GABA by AgRP neurons is required for normal regulation of energy balance. Nat Neurosci. 2008 Sep;11(9):998–1000.
- Campbell JN, Macosko EZ, Fenselau H, Pers TH, Lyubetskaya A, Tenen D, et al. A molecular census of arcuate hypothalamus and median eminence cell types. Nat Neurosci. 2017 Mar;20(3):484–96.
- Fan W, Boston BA, Kesterson RA, Hruby VJ, Cone RD. Role of melanocortinergic neurons in feeding and the agouti obesity syndrome. Nature. 1997 Jan 9;385(6612):165–8.
- Biebermann H, Castañeda TR, van Landeghem F, von Deimling A, Escher F, Brabant G, et al. A role for beta-melanocyte-stimulating hormone in human body-weight regulation. Cell Metab. 2006 Feb;3(2):141–6.
- Lee YS, Challis BG, Thompson DA, Yeo GSH, Keogh JM, Madonna ME, et al. A POMC variant implicates beta-melanocyte-stimulating hormone in the control of human energy balance. Cell Metab. 2006 Feb;3(2):135–40.
- Huszar D, Lynch CA, Fairchild-Huntress V, Dunmore JH, Fang Q, Berkemeier LR, et al. Targeted disruption of the melanocortin-4 receptor results in obesity in mice. Cell. 1997 Jan 10;88(1):131–41.
- Butler AA, Kesterson RA, Khong K, Cullen MJ, Pelleymounter MA, Dekoning J, et al. A unique metabolic syndrome causes obesity in the melanocortin-3 receptor-deficient mouse. Endocrinology. 2000 Sep;141(9):3518–21.
- Chen AS, Marsh DJ, Trumbauer ME, Frazier EG, Guan XM, Yu H, et al. Inactivation of the mouse melanocortin-3 receptor results in increased fat mass and reduced lean body mass. Nat Genet. 2000 Sep;26(1):97–102.
- Sutton GM, Perez-Tilve D, Nogueiras R, Fang J, Kim JK, Cone RD, et al. The melanocortin-3 receptor is required for entrainment to meal intake. J Neurosci. 2008 Nov 26;28(48):12946–55.
- Mountjoy KG, Mortrud MT, Low MJ, Simerly RB, Cone RD. Localization of the melanocortin-4 receptor (MC4-R) in neuroendocrine and autonomic control circuits in the brain. Mol Endocrinol. 1994 Oct;8(10):1298–308.
- Greenfield JR, Miller JW, Keogh JM, Henning E, Satterwhite JH, Cameron GS, et al. Modulation of blood pressure by central melanocortinergic pathways. N Engl J Med. 2009 Jan 1;360(1):44–52.
- Farooqi IS, Keogh JM, Yeo GSH, Lank EJ, Cheetham T, O’Rahilly S. Clinical spectrum of obesity and mutations in the melanocortin 4 receptor gene. N Engl J Med. 2003 Mar 20;348(12):1085–95.
- Farooqi IS, Yeo GS, Keogh JM, Aminian S, Jebb SA, Butler G, et al. Dominant and recessive inheritance of morbid obesity associated with melanocortin 4 receptor deficiency. J Clin Invest. 2000 Jul;106(2):271–9.
- Speliotes EK, Willer CJ, Berndt SI, Monda KL, Thorleifsson G, Jackson AU, et al. Association analyses of 249,796 individuals reveal 18 new loci associated with body mass index. Nat Genet. 2010 Nov;42(11):937–48.
- Balthasar N, Dalgaard LT, Lee CE, Yu J, Funahashi H, Williams T, et al. Divergence of melanocortin pathways in the control of food intake and energy expenditure. Cell. 2005 Nov 4;123(3):493–505.
- Shah BP, Vong L, Olson DP, Koda S, Krashes MJ, Ye C, et al. MC4R-expressing glutamatergic neurons in the paraventricular hypothalamus regulate feeding and are synaptically connected to the parabrachial nucleus. Proc Natl Acad Sci U S A. 2014 Sep 9;111(36):13193–8.
- Cowley MA, Smart JL, Rubinstein M, Cerdán MG, Diano S, Horvath TL, et al. Leptin activates anorexigenic POMC neurons through a neural network in the arcuate nucleus. Nature. 2001 May 24;411(6836):480–4.
- Rau AR, Hentges ST. The Relevance of AgRP Neuron-Derived GABA Inputs to POMC Neurons Differs for Spontaneous and Evoked Release. J Neurosci. 2017 02;37(31):7362–72.
- Atasoy D, Betley JN, Su HH, Sternson SM. Deconstruction of a neural circuit for hunger. Nature. 2012 Aug 9;488(7410):172–7.
- Wu Q, Boyle MP, Palmiter RD. Loss of GABAergic signaling by AgRP neurons to the parabrachial nucleus leads to starvation. Cell. 2009 Jun 26;137(7):1225–34.
- Qin C, Li J, Tang K. The Paraventricular Nucleus of the Hypothalamus: Development, Function, and Human Diseases. Endocrinology. 2018 01;159(9):3458–72.
- Sutton AK, Myers MG, Olson DP. The Role of PVH Circuits in Leptin Action and Energy Balance. Annu Rev Physiol. 2016;78:207–21.
- Holder JL, Butte NF, Zinn AR. Profound obesity associated with a balanced translocation that disrupts the SIM1 gene. Hum Mol Genet. 2000 Jan 1;9(1):101–8.
- Holder JL, Zhang L, Kublaoui BM, DiLeone RJ, Oz OK, Bair CH, et al. Sim1 gene dosage modulates the homeostatic feeding response to increased dietary fat in mice. Am J Physiol Endocrinol Metab. 2004 Jul;287(1):E105-113.
- Garfield AS, Li C, Madara JC, Shah BP, Webber E, Steger JS, et al. A neural basis for melanocortin-4 receptor-regulated appetite. Nat Neurosci. 2015 Jun;18(6):863–71.
- Li MM, Madara JC, Steger JS, Krashes MJ, Balthasar N, Campbell JN, et al. The Paraventricular Hypothalamus Regulates Satiety and Prevents Obesity via Two Genetically Distinct Circuits. Neuron. 2019 May;102(3):653-667.e6.
- Sutton AK, Pei H, Burnett KH, Myers MG, Rhodes CJ, Olson DP. Control of food intake and energy expenditure by Nos1 neurons of the paraventricular hypothalamus. J Neurosci. 2014 Nov 12;34(46):15306–18.
- Blevins JE, Schwartz MW, Baskin DG. Evidence that paraventricular nucleus oxytocin neurons link hypothalamic leptin action to caudal brain stem nuclei controlling meal size. Am J Physiol Regul Integr Comp Physiol. 2004 Jul;287(1):R87-96.
- Kow LM, Pfaff DW. The effects of the TRH metabolite cyclo(His-Pro) and its analogs on feeding. Pharmacol Biochem Behav. 1991 Feb;38(2):359–64.
- Dallman MF, Strack AM, Akana SF, Bradbury MJ, Hanson ES, Scribner KA, et al. Feast and famine: critical role of glucocorticoids with insulin in daily energy flow. Front Neuroendocrinol. 1993 Oct;14(4):303–47.
- Verbalis JG, Blackburn RE, Hoffman GE, Stricker EM. Establishing behavioral and physiological functions of central oxytocin: insights from studies of oxytocin and ingestive behaviors. Adv Exp Med Biol. 1995;395:209–25.
- Britton DR, Koob GF, Rivier J, Vale W. Intraventricular corticotropin-releasing factor enhances behavioral effects of novelty. Life Sci. 1982 Jul 26;31(4):363–7.
- Olson BR, Drutarosky MD, Chow MS, Hruby VJ, Stricker EM, Verbalis JG. Oxytocin and an oxytocin agonist administered centrally decrease food intake in rats. Peptides. 1991 Feb;12(1):113–8.
- Wu Z, Xu Y, Zhu Y, Sutton AK, Zhao R, Lowell BB, et al. An obligate role of oxytocin neurons in diet induced energy expenditure. PLoS One. 2012;7(9):e45167.
- Orthofer M, Valsesia A, Mägi R, Wang Q-P, Kaczanowska J, Kozieradzki I, et al. Identification of ALK in Thinness. Cell. 2020 Jun;181(6):1246-1262.e22.
- Chou TC, Scammell TE, Gooley JJ, Gaus SE, Saper CB, Lu J. Critical role of dorsomedial hypothalamic nucleus in a wide range of behavioral circadian rhythms. J Neurosci. 2003 Nov 19;23(33):10691–702.
- Bellinger LL, Bernardis LL. The dorsomedial hypothalamic nucleus and its role in ingestive behavior and body weight regulation: lessons learned from lesioning studies. Physiol Behav. 2002 Jul;76(3):431–42.
- Patterson CM, Leshan RL, Jones JC, Myers MG. Molecular mapping of mouse brain regions innervated by leptin receptor-expressing cells. Brain Res. 2011 Mar 10;1378:18–28.
- Scott MM, Lachey JL, Sternson SM, Lee CE, Elias CF, Friedman JM, et al. Leptin targets in the mouse brain. J Comp Neurol. 2009 Jun 10;514(5):518–32.
- Rupp AC, Allison MB, Jones JC, Patterson CM, Faber CL, Bozadjieva N, et al. Specific subpopulations of hypothalamic leptin receptor-expressing neurons mediate the effects of early developmental leptin receptor deletion on energy balance. Mol Metab. 2018;14:130–8.
- Rezai-Zadeh K, Yu S, Jiang Y, Laque A, Schwartzenburg C, Morrison CD, et al. Leptin receptor neurons in the dorsomedial hypothalamus are key regulators of energy expenditure and body weight, but not food intake. Mol Metab. 2014 Oct;3(7):681–93.
- Krashes MJ, Shah BP, Madara JC, Olson DP, Strochlic DE, Garfield AS, et al. An excitatory paraventricular nucleus to AgRP neuron circuit that drives hunger. Nature. 2014 Mar;507(7491):238–42.
- Garfield AS, Shah BP, Burgess CR, Li MM, Li C, Steger JS, et al. Dynamic GABAergic afferent modulation of AgRP neurons. Nat Neurosci. 2016;19(12):1628–35.
- Liao G-Y, Kinney CE, An JJ, Xu B. TrkB-expressing neurons in the dorsomedial hypothalamus are necessary and sufficient to suppress homeostatic feeding. Proc Natl Acad Sci U S A. 2019 19;116(8):3256–61.
- Tran PV, Lee MB, Marín O, Xu B, Jones KR, Reichardt LF, et al. Requirement of the orphan nuclear receptor SF-1 in terminal differentiation of ventromedial hypothalamic neurons. Mol Cell Neurosci. 2003 Apr;22(4):441–53.
- Majdic G, Young M, Gomez-Sanchez E, Anderson P, Szczepaniak LS, Dobbins RL, et al. Knockout mice lacking steroidogenic factor 1 are a novel genetic model of hypothalamic obesity. Endocrinology. 2002 Feb;143(2):607–14.
- Dhillon H, Zigman JM, Ye C, Lee CE, McGovern RA, Tang V, et al. Leptin directly activates SF1 neurons in the VMH, and this action by leptin is required for normal body-weight homeostasis. Neuron. 2006 Jan 19;49(2):191–203.
- Hawke Z, Ivanov TR, Bechtold DA, Dhillon H, Lowell BB, Luckman SM. PACAP neurons in the hypothalamic ventromedial nucleus are targets of central leptin signaling. J Neurosci. 2009 Nov 25;29(47):14828–35.
- Borg WP, During MJ, Sherwin RS, Borg MA, Brines ML, Shulman GI. Ventromedial hypothalamic lesions in rats suppress counterregulatory responses to hypoglycemia. J Clin Invest. 1994 Apr;93(4):1677–82.
- Borg WP, Sherwin RS, During MJ, Borg MA, Shulman GI. Local ventromedial hypothalamus glucopenia triggers counterregulatory hormone release. Diabetes. 1995 Feb;44(2):180–4.
- Chan O, Paranjape SA, Horblitt A, Zhu W, Sherwin RS. Lactate-induced release of GABA in the ventromedial hypothalamus contributes to counterregulatory failure in recurrent hypoglycemia and diabetes. Diabetes. 2013 Dec;62(12):4239–46.
- Flak JN, Goforth PB, Dell’Orco J, Sabatini PV, Li C, Bozadjieva N, et al. Ventromedial hypothalamic nucleus neuronal subset regulates blood glucose independently of insulin. Journal of Clinical Investigation. 2020 May 4;130(6):2943–52.
- Qu D, Ludwig DS, Gammeltoft S, Piper M, Pelleymounter MA, Cullen MJ, et al. A role for melanin-concentrating hormone in the central regulation of feeding behaviour. Nature. 1996 Mar 21;380(6571):243–7.
- Shimada M, Tritos NA, Lowell BB, Flier JS, Maratos-Flier E. Mice lacking melanin-concentrating hormone are hypophagic and lean. Nature. 1998 Dec 17;396(6712):670–4.
- de Lecea L, Kilduff TS, Peyron C, Gao X, Foye PE, Danielson PE, et al. The hypocretins: hypothalamus-specific peptides with neuroexcitatory activity. Proc Natl Acad Sci U S A. 1998 Jan 6;95(1):322–7.
- Sakurai T, Amemiya A, Ishii M, Matsuzaki I, Chemelli RM, Tanaka H, et al. Orexins and orexin receptors: a family of hypothalamic neuropeptides and G protein-coupled receptors that regulate feeding behavior. Cell. 1998 Feb 20;92(4):573–85.
- Funato H, Tsai AL, Willie JT, Kisanuki Y, Williams SC, Sakurai T, et al. Enhanced orexin receptor-2 signaling prevents diet-induced obesity and improves leptin sensitivity. Cell Metab. 2009 Jan 7;9(1):64–76.
- de Lecea L, Sutcliffe JG. The hypocretins and sleep. FEBS J. 2005 Nov;272(22):5675–88.
- Louis GW, Leinninger GM, Rhodes CJ, Myers MG. Direct innervation and modulation of orexin neurons by lateral hypothalamic LepRb neurons. J Neurosci. 2010 Aug 25;30(34):11278–87.
- Goforth PB, Leinninger GM, Patterson CM, Satin LS, Myers MG. Leptin acts via lateral hypothalamic area neurotensin neurons to inhibit orexin neurons by multiple GABA-independent mechanisms. J Neurosci. 2014 Aug 20;34(34):11405–15.
- Leinninger GM, Opland DM, Jo Y-H, Faouzi M, Christensen L, Cappellucci LA, et al. Leptin action via neurotensin neurons controls orexin, the mesolimbic dopamine system and energy balance. Cell Metab. 2011 Sep 7;14(3):313–23.
- Leinninger GM, Jo Y-H, Leshan RL, Louis GW, Yang H, Barrera JG, et al. Leptin acts via leptin receptor-expressing lateral hypothalamic neurons to modulate the mesolimbic dopamine system and suppress feeding. Cell Metab. 2009 Aug;10(2):89–98.
- Halaas JL, Gajiwala KS, Maffei M, Cohen SL, Chait BT, Rabinowitz D, et al. Weight-reducing effects of the plasma protein encoded by the obese gene. Science. 1995 Jul 28;269(5223):543–6.
- Pelleymounter MA, Cullen MJ, Baker MB, Hecht R, Winters D, Boone T, et al. Effects of the obese gene product on body weight regulation in ob/ob mice. Science. 1995 Jul 28;269(5223):540–3.
- Campfield LA, Smith FJ, Guisez Y, Devos R, Burn P. Recombinant mouse OB protein: evidence for a peripheral signal linking adiposity and central neural networks. Science. 1995 Jul 28;269(5223):546–9.
- Considine RV, Sinha MK, Heiman ML, Kriauciunas A, Stephens TW, Nyce MR, et al. Serum immunoreactive-leptin concentrations in normal-weight and obese humans. N Engl J Med. 1996 Feb 1;334(5):292–5.
- Frederich RC, Hamann A, Anderson S, Löllmann B, Lowell BB, Flier JS. Leptin levels reflect body lipid content in mice: evidence for diet-induced resistance to leptin action. Nat Med. 1995 Dec;1(12):1311–4.
- Heymsfield SB, Greenberg AS, Fujioka K, Dixon RM, Kushner R, Hunt T, et al. Recombinant leptin for weight loss in obese and lean adults: a randomized, controlled, dose-escalation trial. JAMA. 1999 Oct 27;282(16):1568–75.
- Ahima RS, Saper CB, Flier JS, Elmquist JK. Leptin regulation of neuroendocrine systems. Front Neuroendocrinol. 2000 Jul;21(3):263–307.
- Ahima RS, Prabakaran D, Mantzoros C, Qu D, Lowell B, Maratos-Flier E, et al. Role of leptin in the neuroendocrine response to fasting. Nature. 1996 Jul 18;382(6588):250–2.
- Fujikawa T, Chuang J-C, Sakata I, Ramadori G, Coppari R. Leptin therapy improves insulin-deficient type 1 diabetes by CNS-dependent mechanisms in mice. Proc Natl Acad Sci USA. 2010 Oct 5;107(40):17391–6.
- German JP, Thaler JP, Wisse BE, Oh-I S, Sarruf DA, Matsen ME, et al. Leptin activates a novel CNS mechanism for insulin-independent normalization of severe diabetic hyperglycemia. Endocrinology. 2011 Feb;152(2):394–404.
- Shimomura I, Hammer RE, Ikemoto S, Brown MS, Goldstein JL. Leptin reverses insulin resistance and diabetes mellitus in mice with congenital lipodystrophy. Nature. 1999 Sep 2;401(6748):73–6.
- Morrow T. Myalept approved for treatment of disorders marked by loss of body fat. Manag Care. 2014 Jun;23(6):50–1.
- Cohen P, Zhao C, Cai X, Montez JM, Rohani SC, Feinstein P, et al. Selective deletion of leptin receptor in neurons leads to obesity. J Clin Invest. 2001 Oct;108(8):1113–21.
- Elmquist JK, Bjørbaek C, Ahima RS, Flier JS, Saper CB. Distributions of leptin receptor mRNA isoforms in the rat brain. J Comp Neurol. 1998 Jun 15;395(4):535–47.
- Ring LE, Zeltser LM. Disruption of hypothalamic leptin signaling in mice leads to early-onset obesity, but physiological adaptations in mature animals stabilize adiposity levels. J Clin Invest. 2010 Aug;120(8):2931–41.
- Vong L, Ye C, Yang Z, Choi B, Chua S, Lowell BB. Leptin action on GABAergic neurons prevents obesity and reduces inhibitory tone to POMC neurons. Neuron. 2011 Jul 14;71(1):142–54.
- Leshan RL, Greenwald-Yarnell M, Patterson CM, Gonzalez IE, Myers MG. Leptin action through hypothalamic nitric oxide synthase-1-expressing neurons controls energy balance. Nat Med. 2012 May;18(5):820–3.
- van de Wall E, Leshan R, Xu AW, Balthasar N, Coppari R, Liu SM, et al. Collective and individual functions of leptin receptor modulated neurons controlling metabolism and ingestion. Endocrinology. 2008 Apr;149(4):1773–85.
- Balthasar N, Coppari R, McMinn J, Liu SM, Lee CE, Tang V, et al. Leptin receptor signaling in POMC neurons is required for normal body weight homeostasis. Neuron. 2004 Jun 24;42(6):983–91.
- Xu J, Bartolome CL, Low CS, Yi X, Chien C-H, Wang P, et al. Genetic identification of leptin neural circuits in energy and glucose homeostases. Nature. 2018;556(7702):505–9.
- Kim KW, Zhao L, Donato J, Kohno D, Xu Y, Elias CF, et al. Steroidogenic factor 1 directs programs regulating diet-induced thermogenesis and leptin action in the ventral medial hypothalamic nucleus. Proc Natl Acad Sci U S A. 2011 Jun 28;108(26):10673–8.
- Donato J, Cravo RM, Frazão R, Gautron L, Scott MM, Lachey J, et al. Leptin’s effect on puberty in mice is relayed by the ventral premammillary nucleus and does not require signaling in Kiss1 neurons. J Clin Invest. 2011 Jan;121(1):355–68.
- Robertson SA, Leinninger GM, Myers MG. Molecular and neural mediators of leptin action. Physiol Behav. 2008 Aug 6;94(5):637–42.
- Bates SH, Stearns WH, Dundon TA, Schubert M, Tso AWK, Wang Y, et al. STAT3 signalling is required for leptin regulation of energy balance but not reproduction. Nature. 2003 Feb 20;421(6925):856–9.
- Xu AW, Ste-Marie L, Kaelin CB, Barsh GS. Inactivation of signal transducer and activator of transcription 3 in proopiomelanocortin (Pomc) neurons causes decreased pomc expression, mild obesity, and defects in compensatory refeeding. Endocrinology. 2007 Jan;148(1):72–80.
- Piper ML, Unger EK, Myers MG, Xu AW. Specific physiological roles for signal transducer and activator of transcription 3 in leptin receptor-expressing neurons. Mol Endocrinol. 2008 Mar;22(3):751–9.
- Lee J-Y, Muenzberg H, Gavrilova O, Reed JA, Berryman D, Villanueva EC, et al. Loss of cytokine-STAT5 signaling in the CNS and pituitary gland alters energy balance and leads to obesity. PLoS One. 2008 Feb 20;3(2):e1639.
- Reed JA, Clegg DJ, Smith KB, Tolod-Richer EG, Matter EK, Picard LS, et al. GM-CSF action in the CNS decreases food intake and body weight. J Clin Invest. 2005 Nov;115(11):3035–44.
- Singireddy AV, Inglis MA, Zuure WA, Kim JS, Anderson GM. Neither signal transducer and activator of transcription 3 (STAT3) or STAT5 signaling pathways are required for leptin’s effects on fertility in mice. Endocrinology. 2013 Jul;154(7):2434–45.
- Sipols AJ, Baskin DG, Schwartz MW. Effect of intracerebroventricular insulin infusion on diabetic hyperphagia and hypothalamic neuropeptide gene expression. Diabetes. 1995 Feb;44(2):147–51.
- Brüning JC, Gautam D, Burks DJ, Gillette J, Schubert M, Orban PC, et al. Role of brain insulin receptor in control of body weight and reproduction. Science. 2000 Sep 22;289(5487):2122–5.
- Clegg DJ, Brown LM, Woods SC, Benoit SC. Gonadal hormones determine sensitivity to central leptin and insulin. Diabetes. 2006 Apr;55(4):978–87.
- Könner AC, Janoschek R, Plum L, Jordan SD, Rother E, Ma X, et al. Insulin Action in AgRP-Expressing Neurons Is Required for Suppression of Hepatic Glucose Production. Cell Metabolism. 2007 Jun;5(6):438–49.
- White MF. Insulin signaling in health and disease. Science. 2003 Dec 5;302(5651):1710–1.
- Lin X, Taguchi A, Park S, Kushner JA, Li F, Li Y, et al. Dysregulation of insulin receptor substrate 2 in beta cells and brain causes obesity and diabetes. J Clin Invest. 2004 Oct;114(7):908–16.
- Sadagurski M, Dong XC, Myers MG, White MF. Irs2 and Irs4 synergize in non-LepRb neurons to control energy balance and glucose homeostasis. Mol Metab. 2014 Feb;3(1):55–63.
- Allison MB, Myers MG. 20 years of leptin: connecting leptin signaling to biological function. J Endocrinol. 2014 Oct;223(1):T25-35.
- Klaman LD, Boss O, Peroni OD, Kim JK, Martino JL, Zabolotny JM, et al. Increased energy expenditure, decreased adiposity, and tissue-specific insulin sensitivity in protein-tyrosine phosphatase 1B-deficient mice. Mol Cell Biol. 2000 Aug;20(15):5479–89.
- Zabolotny JM, Bence-Hanulec KK, Stricker-Krongrad A, Haj F, Wang Y, Minokoshi Y, et al. PTP1B regulates leptin signal transduction in vivo. Dev Cell. 2002 Apr;2(4):489–95.
- Banno R, Zimmer D, De Jonghe BC, Atienza M, Rak K, Yang W, et al. PTP1B and SHP2 in POMC neurons reciprocally regulate energy balance in mice. J Clin Invest. 2010 Mar;120(3):720–34.
- Bence KK, Delibegovic M, Xue B, Gorgun CZ, Hotamisligil GS, Neel BG, et al. Neuronal PTP1B regulates body weight, adiposity and leptin action. Nat Med. 2006 Aug;12(8):917–24.
- Loh K, Fukushima A, Zhang X, Galic S, Briggs D, Enriori PJ, et al. Elevated hypothalamic TCPTP in obesity contributes to cellular leptin resistance. Cell Metab. 2011 Nov 2;14(5):684–99.
- Alexander WS, Starr R, Metcalf D, Nicholson SE, Farley A, Elefanty AG, et al. Suppressors of cytokine signaling (SOCS): negative regulators of signal transduction. J Leukoc Biol. 1999 Oct;66(4):588–92.
- Bjorbak C, Lavery HJ, Bates SH, Olson RK, Davis SM, Flier JS, et al. SOCS3 mediates feedback inhibition of the leptin receptor via Tyr985. J Biol Chem. 2000 Dec 22;275(51):40649–57.
- Mori H, Hanada R, Hanada T, Aki D, Mashima R, Nishinakamura H, et al. Socs3 deficiency in the brain elevates leptin sensitivity and confers resistance to diet-induced obesity. Nat Med. 2004 Jul;10(7):739–43.
- Björnholm M, Münzberg H, Leshan RL, Villanueva EC, Bates SH, Louis GW, et al. Mice lacking inhibitory leptin receptor signals are lean with normal endocrine function. J Clin Invest. 2007 May;117(5):1354–60.
- Rui L. SH2B1 regulation of energy balance, body weight, and glucose metabolism. World J Diabetes. 2014 Aug 15;5(4):511–26.
- Ren D, Li M, Duan C, Rui L. Identification of SH2-B as a key regulator of leptin sensitivity, energy balance, and body weight in mice. Cell Metab. 2005 Aug;2(2):95–104.
- Ren D, Zhou Y, Morris D, Li M, Li Z, Rui L. Neuronal SH2B1 is essential for controlling energy and glucose homeostasis. J Clin Invest. 2007 Feb;117(2):397–406.
- Doche ME, Bochukova EG, Su H-W, Pearce LR, Keogh JM, Henning E, et al. Human SH2B1 mutations are associated with maladaptive behaviors and obesity. J Clin Invest. 2012 Dec;122(12):4732–6.
- Jiang L, Su H, Wu X, Shen H, Kim M-H, Li Y, et al. Leptin receptor-expressing neuron Sh2b1 supports sympathetic nervous system and protects against obesity and metabolic disease. Nat Commun. 2020 Dec;11(1):1517.
- Coleman DL, Hummel KP. Effects of parabiosis of normal with genetically diabetic mice. Am J Physiol. 1969 Nov;217(5):1298–304.
- Ravussin Y, Edwin E, Gallop M, Xu L, Bartolomé A, Kraakman MJ, et al. Evidence for a Non-leptin System that Defends against Weight Gain in Overfeeding. Cell Metab. 2018 07;28(2):289-299.e5.
- Qi Y, Takahashi N, Hileman SM, Patel HR, Berg AH, Pajvani UB, et al. Adiponectin acts in the brain to decrease body weight. Nat Med. 2004 May;10(5):524–9.
- Tovar S, Nogueiras R, Tung LYC, Castañeda TR, Vázquez MJ, Morris A, et al. Central administration of resistin promotes short-term satiety in rats. Eur J Endocrinol. 2005 Sep;153(3):R1-5.
- Heppner KM, Tong J, Kirchner H, Nass R, Tschöp MH. The ghrelin O-acyltransferase-ghrelin system: a novel regulator of glucose metabolism. Curr Opin Endocrinol Diabetes Obes. 2011 Feb;18(1):50–5.
- Tschöp M, Smiley DL, Heiman ML. Ghrelin induces adiposity in rodents. Nature. 2000 Oct 19;407(6806):908–13.
- Wren AM, Small CJ, Ward HL, Murphy KG, Dakin CL, Taheri S, et al. The novel hypothalamic peptide ghrelin stimulates food intake and growth hormone secretion. Endocrinology. 2000 Nov;141(11):4325–8.
- Wren AM, Seal LJ, Cohen MA, Brynes AE, Frost GS, Murphy KG, et al. Ghrelin enhances appetite and increases food intake in humans. J Clin Endocrinol Metab. 2001 Dec;86(12):5992.
- Wren AM, Small CJ, Abbott CR, Dhillo WS, Seal LJ, Cohen MA, et al. Ghrelin causes hyperphagia and obesity in rats. Diabetes. 2001 Nov;50(11):2540–7.
- Gottero C, Broglio F, Prodam F, Destefanis S, Bellone S, Benso A, et al. Ghrelin: a link between eating disorders, obesity and reproduction. Nutr Neurosci. 2004 Dec;7(5–6):255–70.
- Yang J, Brown MS, Liang G, Grishin NV, Goldstein JL. Identification of the acyltransferase that octanoylates ghrelin, an appetite-stimulating peptide hormone. Cell. 2008 Feb 8;132(3):387–96.
- Chen HY, Trumbauer ME, Chen AS, Weingarth DT, Adams JR, Frazier EG, et al. Orexigenic action of peripheral ghrelin is mediated by neuropeptide Y and agouti-related protein. Endocrinology. 2004 Jun;145(6):2607–12.
- Cone RD, Cowley MA, Butler AA, Fan W, Marks DL, Low MJ. The arcuate nucleus as a conduit for diverse signals relevant to energy homeostasis. Int J Obes Relat Metab Disord. 2001 Dec;25 Suppl 5:S63-67.
- Castañeda TR, Tong J, Datta R, Culler M, Tschöp MH. Ghrelin in the regulation of body weight and metabolism. Front Neuroendocrinol. 2010 Jan;31(1):44–60.
- Naleid AM, Grace MK, Cummings DE, Levine AS. Ghrelin induces feeding in the mesolimbic reward pathway between the ventral tegmental area and the nucleus accumbens. Peptides. 2005 Nov;26(11):2274–9.
- Faulconbridge LF, Cummings DE, Kaplan JM, Grill HJ. Hyperphagic effects of brainstem ghrelin administration. Diabetes. 2003 Sep;52(9):2260–5.
- Olszewski PK, Grace MK, Billington CJ, Levine AS. Hypothalamic paraventricular injections of ghrelin: effect on feeding and c-Fos immunoreactivity. Peptides. 2003 Jun;24(6):919–23.
- Currie PJ, Mirza A, Fuld R, Park D, Vasselli JR. Ghrelin is an orexigenic and metabolic signaling peptide in the arcuate and paraventricular nuclei. Am J Physiol Regul Integr Comp Physiol. 2005 Aug;289(2):R353–8.
- Flak JN, Myers MG. Minireview: CNS Mechanisms of Leptin Action. Mol Endocrinol. 2016 Jan;30(1):3–12.
- Berthoud H-R, Münzberg H, Morrison CD. Blaming the Brain for Obesity: Integration of Hedonic and Homeostatic Mechanisms. Gastroenterology. 2017;152(7):1728–38.
- Grill HJ, Hayes MR. Hindbrain neurons as an essential hub in the neuroanatomically distributed control of energy balance. Cell Metab. 2012 Sep 5;16(3):296–309.
- Schwartz GJ. Integrative capacity of the caudal brainstem in the control of food intake. Philos Trans R Soc Lond B Biol Sci. 2006 Jul 29;361(1471):1275–80.
- Huo L, Maeng L, Bjørbaek C, Grill HJ. Leptin and the control of food intake: neurons in the nucleus of the solitary tract are activated by both gastric distension and leptin. Endocrinology. 2007 May;148(5):2189–97.
- Huo L, Gamber KM, Grill HJ, Bjørbaek C. Divergent leptin signaling in proglucagon neurons of the nucleus of the solitary tract in mice and rats. Endocrinology. 2008 Feb;149(2):492–7.
- Mason BL, Wang Q, Zigman JM. The central nervous system sites mediating the orexigenic actions of ghrelin. Annu Rev Physiol. 2014;76:519–33.
- Mani BK, Walker AK, Lopez Soto EJ, Raingo J, Lee CE, Perelló M, et al. Neuroanatomical characterization of a growth hormone secretagogue receptor-green fluorescent protein reporter mouse. J Comp Neurol. 2014 Nov 1;522(16):3644–66.
- Hayes MR, Skibicka KP, Leichner TM, Guarnieri DJ, DiLeone RJ, Bence KK, et al. Endogenous Leptin Signaling in the Caudal Nucleus Tractus Solitarius and Area Postrema Is Required for Energy Balance Regulation. Cell Metab. 2016 Apr 12;23(4):744.
- Grill HJ, Schwartz MW, Kaplan JM, Foxhall JS, Breininger J, Baskin DG. Evidence that the caudal brainstem is a target for the inhibitory effect of leptin on food intake. Endocrinology. 2002 Jan;143(1):239–46.
- Kanoski SE, Alhadeff AL, Fortin SM, Gilbert JR, Grill HJ. Leptin signaling in the medial nucleus tractus solitarius reduces food seeking and willingness to work for food. Neuropsychopharmacology. 2014 Feb;39(3):605–13.
- Zsombok A, Jiang Y, Gao H, Anwar IJ, Rezai-Zadeh K, Enix CL, et al. Regulation of leptin receptor-expressing neurons in the brainstem by TRPV1. Physiol Rep. 2014 Sep 1;2(9).
- Yu S, Qualls-Creekmore E, Rezai-Zadeh K, Jiang Y, Berthoud H-R, Morrison CD, et al. Glutamatergic Preoptic Area Neurons That Express Leptin Receptors Drive Temperature-Dependent Body Weight Homeostasis. J Neurosci. 2016 May 4;36(18):5034–46.
- Dodd GT, Worth AA, Nunn N, Korpal AK, Bechtold DA, Allison MB, et al. The thermogenic effect of leptin is dependent on a distinct population of prolactin-releasing peptide neurons in the dorsomedial hypothalamus. Cell Metab. 2014 Oct 7;20(4):639–49.
- Evers SS, Sandoval DA, Seeley RJ. The Physiology and Molecular Underpinnings of the Effects of Bariatric Surgery on Obesity and Diabetes. Annu Rev Physiol. 2017 10;79:313–34.
- Palmiter RD. Neural Circuits That Suppress Appetite: Targets for Treating Obesity? Obesity (Silver Spring). 2017;25(8):1299–301.
- Clemmensen C, Müller TD, Woods SC, Berthoud H-R, Seeley RJ, Tschöp MH. Gut-Brain Cross-Talk in Metabolic Control. Cell. 2017 23;168(5):758–74.
- Adams JM, Pei H, Sandoval DA, Seeley RJ, Chang RB, Liberles SD, et al. Liraglutide Modulates Appetite and Body Weight Through Glucagon-Like Peptide 1 Receptor-Expressing Glutamatergic Neurons. Diabetes. 2018;67(8):1538–48.
- Berthoud HR, Kressel M, Raybould HE, Neuhuber WL. Vagal sensors in the rat duodenal mucosa: distribution and structure as revealed by in vivo DiI-tracing. Anat Embryol (Berl). 1995 Mar;191(3):203–12.
- Williams RM, Berthoud HR, Stead RH. Vagal afferent nerve fibres contact mast cells in rat small intestinal mucosa. Neuroimmunomodulation. 1997 Dec;4(5–6):266–70.
- Kupari J, Häring M, Agirre E, Castelo-Branco G, Ernfors P. An Atlas of Vagal Sensory Neurons and Their Molecular Specialization. Cell Rep. 2019 21;27(8):2508-2523.e4.
- Williams EK, Chang RB, Strochlic DE, Umans BD, Lowell BB, Liberles SD. Sensory Neurons that Detect Stretch and Nutrients in the Digestive System. Cell. 2016 Jun 30;166(1):209–21.
- Bai L, Mesgarzadeh S, Ramesh KS, Huey EL, Liu Y, Gray LA, et al. Genetic Identification of Vagal Sensory Neurons That Control Feeding. Cell. 2019 14;179(5):1129-1143.e23.
- Date Y, Murakami N, Toshinai K, Matsukura S, Niijima A, Matsuo H, et al. The role of the gastric afferent vagal nerve in ghrelin-induced feeding and growth hormone secretion in rats. Gastroenterology. 2002 Oct;123(4):1120–8.
- Patterson LM, Zheng H, Berthoud H-R. Vagal afferents innervating the gastrointestinal tract and CCKA-receptor immunoreactivity. Anat Rec. 2002 01;266(1):10–20.
- Holmes GM, Browning KN, Babic T, Fortna SR, Coleman FH, Travagli RA. Vagal afferent fibres determine the oxytocin-induced modulation of gastric tone. J Physiol. 2013 Jun 15;591(12):3081–100.
- Cork SC, Richards JE, Holt MK, Gribble FM, Reimann F, Trapp S. Distribution and characterisation of Glucagon-like peptide-1 receptor expressing cells in the mouse brain. Mol Metab. 2015 Oct;4(10):718–31.
- Hsu J-Y, Crawley S, Chen M, Ayupova DA, Lindhout DA, Higbee J, et al. Non-homeostatic body weight regulation through a brainstem-restricted receptor for GDF15. Nature. 2017 12;550(7675):255–9.
- Tsai VW-W, Zhang HP, Manandhar R, Schofield P, Christ D, Lee-Ng KKM, et al. GDF15 mediates adiposity resistance through actions on GFRAL neurons in the hindbrain AP/NTS. Int J Obes (Lond). 2019;43(12):2370–80.
- Coester B, Koester-Hegmann C, Lutz TA, Le Foll C. Amylin/Calcitonin Receptor-Mediated Signaling in POMC Neurons Influences Energy Balance and Locomotor Activity in Chow-Fed Male Mice. Diabetes. 2020 Mar 9;db190849.
- Miller AD, Leslie RA. The area postrema and vomiting. Front Neuroendocrinol. 1994 Dec;15(4):301–20.
- Borner T, Shaulson ED, Ghidewon MY, Barnett AB, Horn CC, Doyle RP, et al. GDF15 Induces Anorexia through Nausea and Emesis. Cell Metabolism. 2020 Jan;S1550413119306692.
- Bettge K, Kahle M, Abd El Aziz MS, Meier JJ, Nauck MA. Occurrence of nausea, vomiting and diarrhoea reported as adverse events in clinical trials studying glucagon-like peptide-1 receptor agonists: A systematic analysis of published clinical trials. Diabetes Obes Metab. 2017;19(3):336–47.
- Akaishi T, Takahashi T, Himori N, Fujihara K, Misu T, Abe M, et al. Serum AQP4-IgG level is associated with the phenotype of the first attack in neuromyelitis optica spectrum disorders. J Neuroimmunol. 2020 15;340:577168.
- Zhou C, Liao L, Sun R, Wang J, Di W, Zhu Y, et al. Area postrema syndrome as initial manifestation in neuromyelitis optica spectrum disorder patients: A retrospective study. Rev Neurol (Paris). 2020 Oct 17;
- Chan KH, Vorobeychik G. Area postrema syndrome: a neurological presentation of nausea, vomiting and hiccups. BMJ Case Rep. 2020 Nov 3;13(11).
- Hamilton RB, Norgren R. Central projections of gustatory nerves in the rat. J Comp Neurol. 1984 Feb 1;222(4):560–77.
- Cheng W, Gonzalez I, Pan W, Tsang AH, Adams J, Ndoka E, et al. Calcitonin Receptor Neurons in the Mouse Nucleus Tractus Solitarius Control Energy Balance via the Non-aversive Suppression of Feeding. Cell Metab. 2020 Feb 4;31(2):301-312.e5.
- Cheng W, Ndoka E, Hutch C, Roelofs K, MacKinnon A, Khoury B, et al. Leptin receptor–expressing nucleus tractus solitarius neurons suppress food intake independently of GLP1 in mice. JCI Insight. 2020 Apr 9;5(7):e134359.
- Palmiter RD. The Parabrachial Nucleus: CGRP Neurons Function as a General Alarm. Trends Neurosci. 2018;41(5):280–93.
- Bowen AJ, Chen JY, Huang YW, Baertsch NA, Park S, Palmiter RD. Dissociable control of unconditioned responses and associative fear learning by parabrachial CGRP neurons. Elife. 2020 28;9.
- Campos CA, Bowen AJ, Schwartz MW, Palmiter RD. Parabrachial CGRP Neurons Control Meal Termination. Cell Metab. 2016 May 10;23(5):811–20.
- Little TJ, Horowitz M, Feinle-Bisset C. Role of cholecystokinin in appetite control and body weight regulation. Obes Rev. 2005 Nov;6(4):297–306.
- Kissileff HR, Pi-Sunyer FX, Thornton J, Smith GP. C-terminal octapeptide of cholecystokinin decreases food intake in man. Am J Clin Nutr. 1981 Feb;34(2):154–60.
- Muurahainen N, Kissileff HR, Derogatis AJ, Pi-Sunyer FX. Effects of cholecystokinin-octapeptide (CCK-8) on food intake and gastric emptying in man. Physiol Behav. 1988;44(4–5):645–9.
- Muurahainen NE, Kissileff HR, Lachaussée J, Pi-Sunyer FX. Effect of a soup preload on reduction of food intake by cholecystokinin in humans. Am J Physiol. 1991 Apr;260(4 Pt 2):R672-680.
- Bray GA. Afferent signals regulating food intake. Proc Nutr Soc. 2000 Aug;59(3):373–84.
- Kopin AS, Mathes WF, McBride EW, Nguyen M, Al-Haider W, Schmitz F, et al. The cholecystokinin-A receptor mediates inhibition of food intake yet is not essential for the maintenance of body weight. J Clin Invest. 1999 Feb;103(3):383–91.
- Campbell JE, Drucker DJ. Pharmacology, physiology, and mechanisms of incretin hormone action. Cell Metab. 2013 Jun 4;17(6):819–37.
- Sisley S, Gutierrez-Aguilar R, Scott M, D’Alessio DA, Sandoval DA, Seeley RJ. Neuronal GLP1R mediates liraglutide’s anorectic but not glucose-lowering effect. J Clin Invest. 2014 Jun;124(6):2456–63.
- Drucker DJ. Mechanisms of Action and Therapeutic Application of Glucagon-like Peptide-1. Cell Metab. 2018 03;27(4):740–56.
- Burmeister MA, Ayala JE, Smouse H, Landivar-Rocha A, Brown JD, Drucker DJ, et al. The Hypothalamic Glucagon-Like Peptide 1 Receptor Is Sufficient but Not Necessary for the Regulation of Energy Balance and Glucose Homeostasis in Mice. Diabetes. 2017;66(2):372–84.
- Larsen PJ, Tang-Christensen M, Holst JJ, Orskov C. Distribution of glucagon-like peptide-1 and other preproglucagon-derived peptides in the rat hypothalamus and brainstem. Neuroscience. 1997 Mar;77(1):257–70.
- Parker HE, Reimann F, Gribble FM. Molecular mechanisms underlying nutrient-stimulated incretin secretion. Expert Rev Mol Med. 2010 Jan 5;12:e1.
- Nyberg J, Anderson MF, Meister B, Alborn A-M, Ström A-K, Brederlau A, et al. Glucose-dependent insulinotropic polypeptide is expressed in adult hippocampus and induces progenitor cell proliferation. J Neurosci. 2005 Feb 16;25(7):1816–25.
- Yamada Y, Seino Y. Physiology of GIP--a lesson from GIP receptor knockout mice. Horm Metab Res. 2004 Dec;36(11–12):771–4.
- Wice BM, Wang S, Crimmins DL, Diggs-Andrews KA, Althage MC, Ford EL, et al. Xenin-25 potentiates glucose-dependent insulinotropic polypeptide action via a novel cholinergic relay mechanism. J Biol Chem. 2010 Jun 25;285(26):19842–53.
- Asmar M, Tangaa W, Madsbad S, Hare K, Astrup A, Flint A, et al. On the role of glucose-dependent insulintropic polypeptide in postprandial metabolism in humans. Am J Physiol Endocrinol Metab. 2010 Mar;298(3):E614-621.
- Daousi C, Wilding JPH, Aditya S, Durham BH, Cleator J, Pinkney JH, et al. Effects of peripheral administration of synthetic human glucose-dependent insulinotropic peptide (GIP) on energy expenditure and subjective appetite sensations in healthy normal weight subjects and obese patients with type 2 diabetes. Clin Endocrinol (Oxf). 2009 Aug;71(2):195–201.
- Holst JJ, Rosenkilde MM. GIP as a Therapeutic Target in Diabetes and Obesity: Insight From Incretin Co-agonists. J Clin Endocrinol Metab. 2020 Aug 1;105(8).
- Unsicker K, Spittau B, Krieglstein K. The multiple facets of the TGF-β family cytokine growth/differentiation factor-15/macrophage inhibitory cytokine-1. Cytokine Growth Factor Rev. 2013 Aug;24(4):373–84.
- Fejzo MS, Arzy D, Tian R, MacGibbon KW, Mullin PM. Evidence GDF15 Plays a Role in Familial and Recurrent Hyperemesis Gravidarum. Geburtshilfe Frauenheilkd. 2018 Sep;78(9):866–70.
- Fejzo MS, Fasching PA, Schneider MO, Schwitulla J, Beckmann MW, Schwenke E, et al. Analysis of GDF15 and IGFBP7 in Hyperemesis Gravidarum Support Causality. Geburtshilfe Frauenheilkd. 2019 Apr;79(4):382–8.
- Chrysovergis K, Wang X, Kosak J, Lee S-H, Kim JS, Foley JF, et al. NAG-1/GDF-15 prevents obesity by increasing thermogenesis, lipolysis and oxidative metabolism. Int J Obes (Lond). 2014 Dec;38(12):1555–64.
- Macia L, Tsai VW-W, Nguyen AD, Johnen H, Kuffner T, Shi Y-C, et al. Macrophage inhibitory cytokine 1 (MIC-1/GDF15) decreases food intake, body weight and improves glucose tolerance in mice on normal & obesogenic diets. PLoS One. 2012;7(4):e34868.
- Emmerson PJ, Wang F, Du Y, Liu Q, Pickard RT, Gonciarz MD, et al. The metabolic effects of GDF15 are mediated by the orphan receptor GFRAL. Nat Med. 2017 Oct;23(10):1215–9.
- Yang L, Chang C-C, Sun Z, Madsen D, Zhu H, Padkjær SB, et al. GFRAL is the receptor for GDF15 and is required for the anti-obesity effects of the ligand. Nat Med. 2017 Oct;23(10):1158–66.
- Batterham RL, Bloom SR. The gut hormone peptide YY regulates appetite. Ann N Y Acad Sci. 2003 Jun;994:162–8.
- Batterham RL, Cohen MA, Ellis SM, Le Roux CW, Withers DJ, Frost GS, et al. Inhibition of food intake in obese subjects by peptide YY3-36. N Engl J Med. 2003 Sep 4;349(10):941–8.
- Batterham RL, Cowley MA, Small CJ, Herzog H, Cohen MA, Dakin CL, et al. Gut hormone PYY(3-36) physiologically inhibits food intake. Nature. 2002 Aug 8;418(6898):650–4.
- Grandt D, Schimiczek M, Beglinger C, Layer P, Goebell H, Eysselein VE, et al. Two molecular forms of peptide YY (PYY) are abundant in human blood: characterization of a radioimmunoassay recognizing PYY 1-36 and PYY 3-36. Regul Pept. 1994 May 5;51(2):151–9.
- Nonaka N, Shioda S, Niehoff ML, Banks WA. Characterization of blood-brain barrier permeability to PYY3-36 in the mouse. J Pharmacol Exp Ther. 2003 Sep;306(3):948–53.
- Boggiano MM, Chandler PC, Oswald KD, Rodgers RJ, Blundell JE, Ishii Y, et al. PYY3-36 as an anti-obesity drug target. Obes Rev. 2005 Nov;6(4):307–22.
- Tschöp M, Castañeda TR, Joost HG, Thöne-Reineke C, Ortmann S, Klaus S, et al. Physiology: does gut hormone PYY3-36 decrease food intake in rodents? Nature. 2004 Jul 8;430(6996):1 p following 165; discussion 2 p following 165.
- Halatchev IG, Cone RD. Peripheral administration of PYY(3-36) produces conditioned taste aversion in mice. Cell Metab. 2005 Mar;1(3):159–68.
- Pittner RA, Moore CX, Bhavsar SP, Gedulin BR, Smith PA, Jodka CM, et al. Effects of PYY[3-36] in rodent models of diabetes and obesity. Int J Obes Relat Metab Disord. 2004 Aug;28(8):963–71.
- Chance WT, Balasubramaniam A, Zhang FS, Wimalawansa SJ, Fischer JE. Anorexia following the intrahypothalamic administration of amylin. Brain Res. 1991 Jan 25;539(2):352–4.
- Lutz TA, Del Prete E, Scharrer E. Reduction of food intake in rats by intraperitoneal injection of low doses of amylin. Physiol Behav. 1994 May;55(5):891–5.
- Ludvik B, Kautzky-Willer A, Prager R, Thomaseth K, Pacini G. Amylin: history and overview. Diabet Med. 1997 Jun;14 Suppl 2:S9-13.
- Rushing PA, Hagan MM, Seeley RJ, Lutz TA, Woods SC. Amylin: a novel action in the brain to reduce body weight. Endocrinology. 2000 Feb;141(2):850–3.
- Christopoulos G, Perry KJ, Morfis M, Tilakaratne N, Gao Y, Fraser NJ, et al. Multiple amylin receptors arise from receptor activity-modifying protein interaction with the calcitonin receptor gene product. Mol Pharmacol. 1999 Jul;56(1):235–42.
- Roth JD, Roland BL, Cole RL, Trevaskis JL, Weyer C, Koda JE, et al. Leptin responsiveness restored by amylin agonism in diet-induced obesity: evidence from nonclinical and clinical studies. Proc Natl Acad Sci U S A. 2008 May 20;105(20):7257–62.
- D’Agostino G, Lyons DJ, Cristiano C, Burke LK, Madara JC, Campbell JN, et al. Appetite controlled by a cholecystokinin nucleus of the solitary tract to hypothalamus neurocircuit. Elife. 2016 14;5.
- Mayer J. Regulation of energy intake and the body weight: the glucostatic theory and the lipostatic hypothesis. Ann N Y Acad Sci. 1955 Jul 15;63(1):15–43.
- Mayer J, Thomas DW. Regulation of food intake and obesity. Science. 1967 Apr 21;156(3773):328–37.
- Mountjoy PD, Rutter GA. Glucose sensing by hypothalamic neurones and pancreatic islet cells: AMPle evidence for common mechanisms? Exp Physiol. 2007 Mar;92(2):311–9.
- McCrimmon RJ, Fan X, Ding Y, Zhu W, Jacob RJ, Sherwin RS. Potential role for AMP-activated protein kinase in hypoglycemia sensing in the ventromedial hypothalamus. Diabetes. 2004 Aug;53(8):1953–8.
- Han S-M, Namkoong C, Jang PG, Park IS, Hong SW, Katakami H, et al. Hypothalamic AMP-activated protein kinase mediates counter-regulatory responses to hypoglycaemia in rats. Diabetologia. 2005 Oct;48(10):2170–8.
- Lam TKT. Regulation of Blood Glucose by Hypothalamic Pyruvate Metabolism. Science. 2005 Aug 5;309(5736):943–7.
- Villanueva EC, Münzberg H, Cota D, Leshan RL, Kopp K, Ishida-Takahashi R, et al. Complex regulation of mammalian target of rapamycin complex 1 in the basomedial hypothalamus by leptin and nutritional status. Endocrinology. 2009 Oct;150(10):4541–51.
- White BD, Du F, Higginbotham DA. Low dietary protein is associated with an increase in food intake and a decrease in the in vitro release of radiolabeled glutamate and GABA from the lateral hypothalamus. Nutr Neurosci. 2003 Dec;6(6):361–7.
- Heeley N, Blouet C. Central Amino Acid Sensing in the Control of Feeding Behavior. Front Endocrinol (Lausanne). 2016;7:148.
- Hu F, Xu Y, Liu F. Hypothalamic roles of mTOR complex I: integration of nutrient and hormone signals to regulate energy homeostasis. Am J Physiol Endocrinol Metab. 2016 01;310(11):E994–1002.
- Vandenbeuch A, Kinnamon SC. Glutamate: Tastant and Neuromodulator in Taste Buds. Adv Nutr. 2016 Jul;7(4):823S-827S.
- Kondoh T, Mallick HN, Torii K. Activation of the gut-brain axis by dietary glutamate and physiologic significance in energy homeostasis. Am J Clin Nutr. 2009 Sep;90(3):832S-837S.
- Greenberg AS, Obin MS. Obesity and the role of adipose tissue in inflammation and metabolism. Am J Clin Nutr. 2006 Feb;83(2):461S-465S.
- Marks DL, Ling N, Cone RD. Role of the central melanocortin system in cachexia. Cancer Res. 2001 Feb 15;61(4):1432–8.
- Yao JH, Ye SM, Burgess W, Zachary JF, Kelley KW, Johnson RW. Mice deficient in interleukin-1beta converting enzyme resist anorexia induced by central lipopolysaccharide. Am J Physiol. 1999;277(5):R1435-1443.
- Dong ZM, Gutierrez-Ramos JC, Coxon A, Mayadas TN, Wagner DD. A new class of obesity genes encodes leukocyte adhesion receptors. Proc Natl Acad Sci U S A. 1997 Jul 8;94(14):7526–30.
- Hirsch E, Irikura VM, Paul SM, Hirsh D. Functions of interleukin 1 receptor antagonist in gene knockout and overproducing mice. Proc Natl Acad Sci U S A. 1996 Oct 1;93(20):11008–13.
- Hotamisligil GS, Shargill NS, Spiegelman BM. Adipose expression of tumor necrosis factor-alpha: direct role in obesity-linked insulin resistance. Science. 1993 Jan 1;259(5091):87–91.
- Hotamisligil GS. The role of TNFalpha and TNF receptors in obesity and insulin resistance. J Intern Med. 1999 Jun;245(6):621–5.
- Weisberg SP, McCann D, Desai M, Rosenbaum M, Leibel RL, Ferrante AW. Obesity is associated with macrophage accumulation in adipose tissue. J Clin Invest. 2003 Dec;112(12):1796–808.
- Lumeng CN, Saltiel AR. Inflammatory links between obesity and metabolic disease. J Clin Invest. 2011 Jun;121(6):2111–7.
- Ferrante AW. The immune cells in adipose tissue. Diabetes Obes Metab. 2013 Sep;15 Suppl 3:34–8.
- Gao Y, Tschöp MH, Luquet S. Hypothalamic tanycytes: gatekeepers to metabolic control. Cell Metab. 2014 Feb 4;19(2):173–5.
- Thaler JP, Yi C-X, Schur EA, Guyenet SJ, Hwang BH, Dietrich MO, et al. Obesity is associated with hypothalamic injury in rodents and humans. J Clin Invest. 2012 Jan;122(1):153–62.
- Ozcan L, Ergin AS, Lu A, Chung J, Sarkar S, Nie D, et al. Endoplasmic reticulum stress plays a central role in development of leptin resistance. Cell Metab. 2009 Jan 7;9(1):35–51.
- Rolls ET. Taste, olfactory, and food texture processing in the brain, and the control of food intake. Physiol Behav. 2005 May 19;85(1):45–56.
- Rolls ET. Sensory processing in the brain related to the control of food intake. Proc Nutr Soc. 2007 Feb;66(1):96–112.
- Mahler SV, Smith KS, Berridge KC. Endocannabinoid hedonic hotspot for sensory pleasure: anandamide in nucleus accumbens shell enhances “liking” of a sweet reward. Neuropsychopharmacology. 2007 Nov;32(11):2267–78.
- Peciña S, Smith KS, Berridge KC. Hedonic hot spots in the brain. Neuroscientist. 2006 Dec;12(6):500–11.
- Smith KS, Berridge KC. Opioid limbic circuit for reward: interaction between hedonic hotspots of nucleus accumbens and ventral pallidum. J Neurosci. 2007 Feb 14;27(7):1594–605.
- Robinson S, Sotak BN, During MJ, Palmiter RD. Local dopamine production in the dorsal striatum restores goal-directed behavior in dopamine-deficient mice. Behav Neurosci. 2006 Feb;120(1):196–200.
- Marston OJ, Garfield AS, Heisler LK. Role of central serotonin and melanocortin systems in the control of energy balance. Eur J Pharmacol. 2011 Jun 11;660(1):70–9.
- Sohn J-W, Xu Y, Jones JE, Wickman K, Williams KW, Elmquist JK. Serotonin 2C receptor activates a distinct population of arcuate pro-opiomelanocortin neurons via TRPC channels. Neuron. 2011 Aug 11;71(3):488–97.
- Heisler LK, Cowley MA, Tecott LH, Fan W, Low MJ, Smart JL, et al. Activation of central melanocortin pathways by fenfluramine. Science. 2002 Jul 26;297(5581):609–11.
- Kozak LP, Harper ME. Mitochondrial uncoupling proteins in energy expenditure. Annu Rev Nutr. 2000;20:339–63.
- Lowell BB, S-Susulic V, Hamann A, Lawitts JA, Himms-Hagen J, Boyer BB, et al. Development of obesity in transgenic mice after genetic ablation of brown adipose tissue. Nature. 1993 Dec 23;366(6457):740–2.
- Bachman ES, Dhillon H, Zhang C-Y, Cinti S, Bianco AC, Kobilka BK, et al. betaAR signaling required for diet-induced thermogenesis and obesity resistance. Science. 2002 Aug 2;297(5582):843–5.
- Vaisse C, Clement K, Guy-Grand B, Froguel P. A frameshift mutation in human MC4R is associated with a dominant form of obesity. Nat Genet. 1998 Oct;20(2):113–4.
- Loos RJF, Lindgren CM, Li S, Wheeler E, Zhao JH, Prokopenko I, et al. Common variants near MC4R are associated with fat mass, weight and risk of obesity. Nat Genet. 2008 Jun;40(6):768–75.
- O’Rahilly S, Farooqi IS. Human obesity as a heritable disorder of the central control of energy balance. Int J Obes (Lond). 2008 Dec;32 Suppl 7:S55-61.
- Kühnen P, Clément K, Wiegand S, Blankenstein O, Gottesdiener K, Martini LL, et al. Proopiomelanocortin Deficiency Treated with a Melanocortin-4 Receptor Agonist. N Engl J Med. 2016 Jul 21;375(3):240–6.
- Collet T-H, Dubern B, Mokrosinski J, Connors H, Keogh JM, Mendes de Oliveira E, et al. Evaluation of a melanocortin-4 receptor (MC4R) agonist (Setmelanotide) in MC4R deficiency. Molecular Metabolism. 2017 Oct;6(10):1321–9.
- Montague CT, Farooqi IS, Whitehead JP, Soos MA, Rau H, Wareham NJ, et al. Congenital leptin deficiency is associated with severe early-onset obesity in humans. Nature. 1997 Jun 26;387(6636):903–8.
- Farooqi IS, Jebb SA, Langmack G, Lawrence E, Cheetham CH, Prentice AM, et al. Effects of recombinant leptin therapy in a child with congenital leptin deficiency. N Engl J Med. 1999 Sep 16;341(12):879–84.
- Clément K, Vaisse C, Lahlou N, Cabrol S, Pelloux V, Cassuto D, et al. A mutation in the human leptin receptor gene causes obesity and pituitary dysfunction. Nature. 1998 Mar 26;392(6674):398–401.
- Chung WK, Belfi K, Chua M, Wiley J, Mackintosh R, Nicolson M, et al. Heterozygosity for Lep(ob) or Lep(rdb) affects body composition and leptin homeostasis in adult mice. Am J Physiol. 1998;274(4):R985-990.
- Farooqi IS, Wangensteen T, Collins S, Kimber W, Matarese G, Keogh JM, et al. Clinical and molecular genetic spectrum of congenital deficiency of the leptin receptor. N Engl J Med. 2007 Jan 18;356(3):237–47.
- Blüher S, Shah S, Mantzoros CS. Leptin deficiency: clinical implications and opportunities for therapeutic interventions. J Investig Med. 2009 Oct;57(7):784–8.
- Sharma N, Berbari NF, Yoder BK. Ciliary dysfunction in developmental abnormalities and diseases. Curr Top Dev Biol. 2008;85:371–427.
- Zaghloul NA, Katsanis N. Mechanistic insights into Bardet-Biedl syndrome, a model ciliopathy. J Clin Invest. 2009 Mar;119(3):428–37.
- Jackson RS, Creemers JW, Ohagi S, Raffin-Sanson ML, Sanders L, Montague CT, et al. Obesity and impaired prohormone processing associated with mutations in the human prohormone convertase 1 gene. Nat Genet. 1997 Jul;16(3):303–6.
- Alsters SIM, Goldstone AP, Buxton JL, Zekavati A, Sosinsky A, Yiorkas AM, et al. Truncating Homozygous Mutation of Carboxypeptidase E (CPE) in a Morbidly Obese Female with Type 2 Diabetes Mellitus, Intellectual Disability and Hypogonadotrophic Hypogonadism. PLoS One. 2015;10(6):e0131417.
- Greenberg ME, Xu B, Lu B, Hempstead BL. New insights in the biology of BDNF synthesis and release: implications in CNS function. J Neurosci. 2009 Oct 14;29(41):12764–7.
- Gray J, Yeo GSH, Cox JJ, Morton J, Adlam A-LR, Keogh JM, et al. Hyperphagia, severe obesity, impaired cognitive function, and hyperactivity associated with functional loss of one copy of the brain-derived neurotrophic factor (BDNF) gene. Diabetes. 2006 Dec;55(12):3366–71.
- Han JC, Liu Q-R, Jones M, Levinn RL, Menzie CM, Jefferson-George KS, et al. Brain-derived neurotrophic factor and obesity in the WAGR syndrome. N Engl J Med. 2008 Aug 28;359(9):918–27.
- Gray J, Yeo G, Hung C, Keogh J, Clayton P, Banerjee K, et al. Functional characterization of human NTRK2 mutations identified in patients with severe early-onset obesity. Int J Obes (Lond). 2007 Feb;31(2):359–64.
- Mercader JM, Saus E, Agüera Z, Bayés M, Boni C, Carreras A, et al. Association of NTRK3 and its interaction with NGF suggest an altered cross-regulation of the neurotrophin signaling pathway in eating disorders. Hum Mol Genet. 2008 May 1;17(9):1234–44.
- Kernie SG, Liebl DJ, Parada LF. BDNF regulates eating behavior and locomotor activity in mice. EMBO J. 2000 Mar 15;19(6):1290–300.
- Unger TJ, Calderon GA, Bradley LC, Sena-Esteves M, Rios M. Selective deletion of Bdnf in the ventromedial and dorsomedial hypothalamus of adult mice results in hyperphagic behavior and obesity. J Neurosci. 2007 Dec 26;27(52):14265–74.
- Buiting K. Prader-Willi syndrome and Angelman syndrome. Am J Med Genet C Semin Med Genet. 2010 Aug 15;154C(3):365–76.
- Whittington J, Holland A. Neurobehavioral phenotype in Prader-Willi syndrome. Am J Med Genet C Semin Med Genet. 2010 Nov 15;154C(4):438–47.
- Ding F, Li HH, Zhang S, Solomon NM, Camper SA, Cohen P, et al. SnoRNA Snord116 (Pwcr1/MBII-85) deletion causes growth deficiency and hyperphagia in mice. PLoS One. 2008 Mar 5;3(3):e1709.
- Bischof JM, Stewart CL, Wevrick R. Inactivation of the mouse Magel2 gene results in growth abnormalities similar to Prader-Willi syndrome. Hum Mol Genet. 2007 Nov 15;16(22):2713–9.
- Mercer RE, Kwolek EM, Bischof JM, van Eede M, Henkelman RM, Wevrick R. Regionally reduced brain volume, altered serotonin neurochemistry, and abnormal behavior in mice null for the circadian rhythm output gene Magel2. Am J Med Genet B Neuropsychiatr Genet. 2009 Dec 5;150B(8):1085–99.
- Muscatelli F, Abrous DN, Massacrier A, Boccaccio I, Le Moal M, Cau P, et al. Disruption of the mouse Necdin gene results in hypothalamic and behavioral alterations reminiscent of the human Prader-Willi syndrome. Hum Mol Genet. 2000 Dec 12;9(20):3101–10.